Understanding Photoluminescence: Concepts and Applications
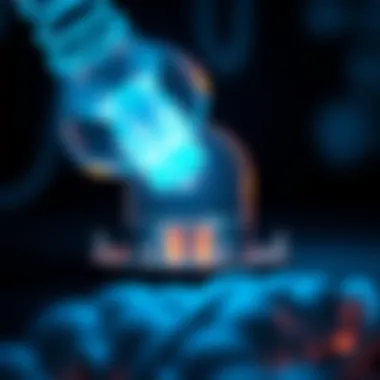
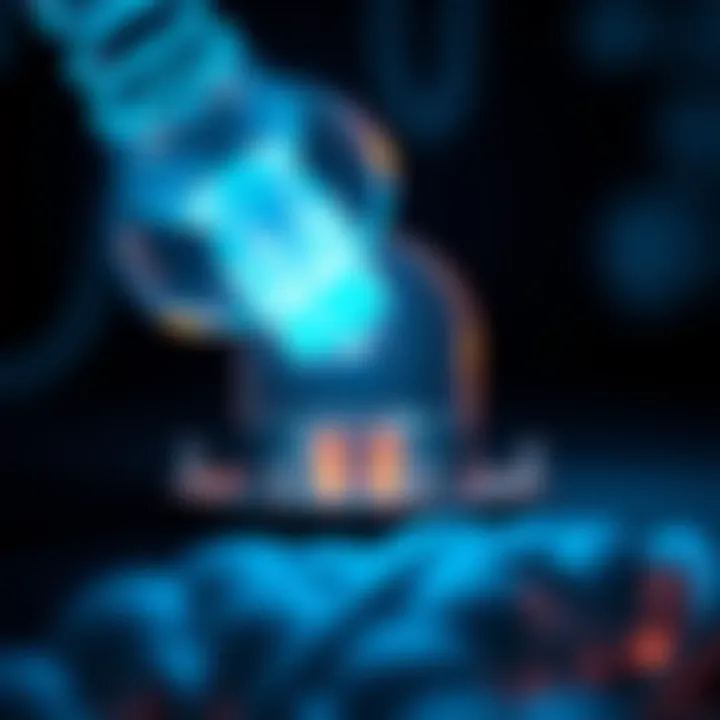
Intro
Photoluminescence is a fascinating physical phenomenon that has captured the attention of scientists and researchers for many years. It involves the absorption of photons or light, which then leads to the re-emission of light. In essence, when a material absorbs energy, it typically does so in the form of light. This absorbed energy excites electrons within atoms, elevating them to a higher energy state. Once they return to their original state, they release energy in the form of light, explicating the core principle of photoluminescence.
This article sets out to provide a detailed examination of photoluminescence, not only delving into its fundamental concepts but also illuminating the various applications across fields like materials science, biology, and nanotechnology. By unpacking the differences between fluorescence and phosphorescence, we aim to underscore the significance of these phenomena in scientific research and various industries.
In today’s science-driven world, understanding the mechanics of photoluminescence becomes vital for both researchers and professionals. As technology advances, the application of photoluminescent materials has been growing rapidly, promising new avenues in technology and science. This exploration will highlight not only the theoretical underpinnings of photoluminescence but also its practical implications, including innovative advancements in materials.
Overview of Research Topic
Brief Background and Context
Photoluminescence has roots in the early explorations of light and energy conversion processes. Scientific advancements in understanding atomic and molecular structures have paved the way for breakthroughs in this field. Origins trace back to discoveries made in the 19th century, yet the industrial application of photoluminescent materials is a relatively recent endeavor. Today, research involves an array of luminescent materials that display various properties depending on their composition and structure.
Also noteworthy is the growing interest in using photoluminescence as an analytical tool. No longer confined to academic settings, its uses span from diagnostics in biological systems to quality control in manufacturing processes.
Importance in Current Scientific Landscape
The relevance of photoluminescence within the scientific community cannot be overstated. As industries adapt to technological advancements, the ability to harness light for practical application becomes crucial.
- In materials science, photoluminescent materials serve a fundamental role in the development of new compounds, with applications ranging from LEDs to solar cells.
- In the biological field, the ability to trace metabolic pathways and cellular processes has created a niche where photoluminescence enables researchers to visualize complex interactions in living systems.
- The rise of nanotechnology showcases how photoluminescent materials can be manipulated at the nanoscale, allowing for innovative designs in medicine and electronics.
As we further explore the specifics of photoluminescence, it becomes evident that its influence touches nearly every corner of modern science and technology. The implications of recent advancements lay a foundation for future breakthroughs that could very well redefine industries.
“Understanding photoluminescence is crucial for advancing various fields, making it a key focus for researchers and professionals alike.”
Prolusion to Photoluminescence
Photoluminescence is a fascinating area within the study of light-matter interactions, crucial to understanding how materials respond to varied light stimuli. This phenomenon provides profound insights not only into fundamental physics but also into applied science, revealing the inner workings of atomic and molecular processes. By investigating how substances absorb photons and subsequently re-emit them, researchers unlock doors to a multitude of applications in fields such as materials science, biology, and nanotechnology.
One significant benefit of studying photoluminescence lies in its broad applicability. For instance, photoluminescent materials are at the forefront of advancements in optoelectronic devices, sensors, and imaging technology. Moreover, understanding these processes allows scientists to engineer novel materials with specific characteristics that have implications in renewable energy, electronics, and even medical diagnostics.
Key considerations regarding photoluminescence include the factors that influence its efficiency, the types of materials that exhibit it, and the underlying mechanisms governing its behavior. Addressing these elements is not merely academic; it can lead to practical enhancements in existing technologies, making the exploration of photoluminescence transcend theoretical limits and find utility in everyday applications.
In essence, photoluminescence bridges the gap between science and technology, making it a pivotal subject in contemporary research and innovation.
Definition and Basics
At its core, photoluminescence refers to the emission of light (or luminescence) from a material after it has absorbed photons. When light hits a photoluminescent material, its atoms or molecules are excited to higher energy states. Once they return to their original state, they release energy in the form of light. This process not only sheds light on the material's electronic structure but also provides a diagnostic tool for characterizing materials.
The basic framework of photoluminescence can be divided into two main stages: excitation and emission. During excitation, the energy from photons is absorbed, promoting electrons to higher energy levels. The subsequent emission occurs as these excited electrons drop back to lower energy states, releasing the absorbed energy as light.
Historical Context
The exploration of photoluminescence can be traced back to the late 19th century when scientists began to uncover the principles of light absorption and emission. Influential figures such as Heinrich Hertz and Wilhelm Röntgen laid the foundation for understanding light-matter interactions, which would later evolve into the complex field of photoluminescence.
Despite its relatively recent discovery, the significance of photoluminescence gained momentum in the 20th century, particularly with the development of quantum mechanics. Researchers like Niels Bohr and Albert Einstein contributed to the comprehension of energy states and the behavior of photons, further enhancing the understanding of photoluminescent materials.
With advancements in technology, especially in spectroscopy and microscopy, the capabilities to measure and manipulate photoluminescent properties have expanded, making it an essential tool in both academic and industrial research today.
Fundamental Mechanisms
Understanding the fundamental mechanisms of photoluminescence is essential for grasping how this phenomenon operates and its implications across various fields. The excitation and emission processes form the backbone of photoluminescence. By diving into these specifics, one can appreciate not just the science but also the practical applications that arise from them. Grasping these mechanisms allows researchers and technologists to tailor materials for specific usages, enhancing their efficiency and effectiveness. Therefore, a detailed exploration of these elements provides insight into the broader impact of photoluminescent materials in contemporary science and technology.
Excitation Process
The excitation process is the first step in the photoluminescent journey. Simply put, it is when an atom or a molecule absorbs energy, usually from light, causing an electron to jump from a lower energy level to a higher one. This transition is not just a matter of energy; it’s akin to filling up a jug with water—the water representing the energy and the jug the electron's capacity to hold it.
Different materials exhibit distinct excitation energies, which can greatly affect their luminescent properties. For example, in semiconductors, the excitation can occur at various bandwidths, which in turn influences the emitted light's color—a critical aspect for applications like LEDs and lasers.
Key Points on Excitation:
- Energy Absorption: The energy must equal or exceed the material's band gap.
- Duration of Excitation: The length of the excitation can impact how long the resulting emission will last.
- Environmental Factors: Temperature and surrounding materials can change how effective the excitation is.
Emission Process
Once an electron is excited, it doesn’t just stay there forever. Eventually, it will lose energy and the electron falls back to its original state, releasing the absorbed energy in the form of light. This is the emission process. It can be thought of as a bouncy ball that goes up high but eventually returns to its starting point. The light emitted can vary significantly, depending on how the electron behaves while returning.
There are various factors that influence this process:
- Relaxation Time: This is the time it takes for the excited state to lose energy before emitting light. Different materials can have different relaxation times, leading to various forms of luminescence.
- Quantum Yield: This is a measure of how many photons are emitted for every photon absorbed. A high quantum yield means a brighter emitted light, which is vital in many applications, particularly in biomedical imaging.
Energy States Involved
Understanding the energy states involved in photoluminescence is fundamental. When the electron absorbs energy, it transitions to an excited state. Once it releases energy, it returns to a lower energy state. The interplay between these states—specifically, the conduction band, valence band, and the energy levels within them—determines the properties of the emitted light.
- Conduction Band: This is where the electrons move freely and can contribute to conduction in materials.
- Valence Band: Electrons in this band are bound to atoms and do not contribute to electrical conductivity.
- Trap States: Materials can have localized energy states, often leading to phenomena like phosphorescence where light is emitted long after the excitation source is removed.
Understanding these energy states can lead to advancements in creating new materials that exhibit specific luminescent properties, impacting various technological fields like electronics and photonics.
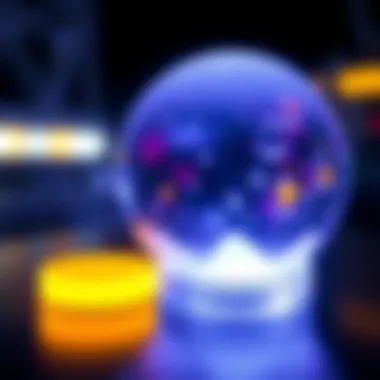
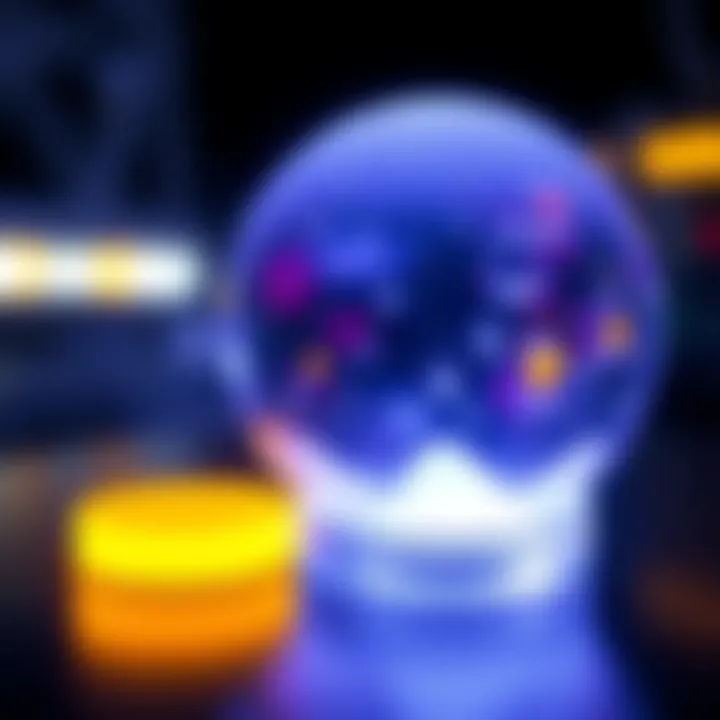
In sum, the excitation and emission processes, along with the energy states involved, lay the groundwork for understanding photoluminescence. Mastering these concepts is not just academic; it has real-world ramifications, influencing innovations in countless applications.
Types of Photoluminescence
Photoluminescence, with its layered intricacies, stands as a significant topic when one considers its varied applications in science and technology. Understanding different types of photoluminescence not only lays the foundation for further exploration in fields like materials science and bioengineering, it also shines a spotlight on how we harness light for various functionalities. Let’s look into the two primary types: fluorescence and phosphorescence. Both are prevalent, yet they possess unique attributes that serve distinct purposes.
Fluorescence
Fluorescence is perhaps the more familiar of the two. It occurs when a substance absorbs photons and then re-emits them almost instantaneously, often within nanoseconds. This quick return to the ground state distinguishes fluorescence from other luminescent processes. The brief nature of fluorescence makes it tremendously useful in many practical applications, such as:
- Biological staining: Fluorescent dyes can label biological tissues, making it easier to examine cellular structures under a microscope.
- Fluorescent markers in research: Many scientists use fluorescent proteins to visualize cells in real time, providing insight into processes such as protein interactions.
- Safety signage: Brightly colored fluorescent materials are used in signs and apparel, making them more visible in low-light conditions.
The underlying mechanisms of fluorescence can be quite intricate, involving electronic transitions that happen in distinct energy states. After absorbing energy, an electron gets excited to a higher energy level before dropping back down and releasing energy as a photon. The wavelength of this emitted light tends to be longer than that of the absorbed one, which is a key principle used in various equipment like fluorometers.
"In the realm of photoluminescence, fluorescence paves the path for countless advances in diagnostics and imaging, relatively easily observed in everyday applications."
Phosphorescence
Phosphorescence, while related to fluorescence, has its own charm. It can be thought of as a slower cousin—sometimes seen in glow-in-the-dark materials. The crux of phosphorescence is its prolonged duration; when excited, a material can store energy for a longer time before re-emitting it, sometimes lasting from milliseconds to hours.
This delayed emission stems from the involvement of "triplet" energy states, which are less likely to return to their ground state immediately. This characteristic allows phosphorescent materials to hold onto their glow even after the excitation source is removed. Applications that leverage this unique property include:
- Emergency signs: Phosphorescent materials illuminate pathways when the lights go out, guiding individuals safely to exits during emergencies.
- Decorative items: Glow-in-the-dark stars and wall decals that provide a whimsical ambiance in children's rooms.
- Security inks: Certain inks used in product packaging can fluoresce under normal light but remain visible longer in darkness, helping in anti-counterfeiting measures.
Understanding the differences between fluorescence and phosphorescence showcases the versatility of photoluminescent materials. Each type offers distinct benefits that cater to different needs across various industries. As we delve deeper into the realm of photoluminescence, keeping these differences in mind becomes essential for anyone engaged in related research or applications.
Measuring Photoluminescence
Measuring photoluminescence is a cornerstone in understanding the properties and behaviors of luminescent materials. This process provides valuable insights into material characteristics, efficiency, and overall performance. Understanding how to precisely measure photoluminescence allows researchers and industry professionals alike to optimize applications in various fields, such as optoelectronics, nanotechnology, and biological imaging. Accurate measurements can lead to enhanced material development, efficient diagnostics, and innovative technologies.
Techniques and Equipment
The measurement of photoluminescence relies on several sophisticated techniques and equipment. Among them, the most common methods include:
- Spectroscopy: This is the primary technique used to analyze the emitted light spectrum after excitation. Devices such as photoluminescence spectrometers capture emitted photons, often revealing intricate details about electronic structure and energy levels.
- Cryogenic Systems: Using cryogenic conditions can enhance the performance of many luminescent materials. Cooling down samples minimizes thermal noise, leading to more precise readings. For instance, a closed-cycle cryostat allows for stable temperature management during experiments.
- Laser Excitation Sources: Lasers provide the utmost control over the excitation energy being applied to the sample. Different lasers might be selected based on the required energy level, thus tailoring the investigation to the material in question.
- Charge-Coupled Devices (CCDs): CCDs serve as highly sensitive detectors that capture and convert emitted light to digital signals. This enables detailed analysis of the spectroscopic data collected.
Facilitating effective measurement may require knowledge about specific setups. The calibration of instruments, understanding limitations based on the properties of the materials, and tailoring configurations to optimize signal-to-noise ratios are significant factors.
Analysis and Interpretation of Data
Once photoluminescence measurements are obtained, the next step involves analyzing and interpreting the data accurately. Researchers should be well-versed in a few essential aspects in this process:
- Spectral Shape: The shape of the photoluminescence spectra can indicate underlying mechanisms of emission such as recombination processes or energy transfer phenomena. Sharp peaks may correlate with distinct transitions while broad backgrounds may provide insight into defect states within the materials.
- Quantum Yield Calculation: Quantum yield describes the efficiency of photoluminescence, defined as the ratio of emitted photons to absorbed photons. A higher quantum yield indicates more efficient material, which is particularly critical in applications like light-emitting diodes or solar cells.
- Temperature Dependence: Analyzing how photoluminescence shifts with temperature can unveil details about mobility and defect levels. Plots that demonstrate intensity or peaks shifting can help understand how temperature affects excited states within the material.
- Lifetime Measurements: Determining the time an excited state persists before emitting light can be crucial. Techniques like time-correlated single photon counting provide this data, allowing researchers to investigate energy transfer mechanisms as well as interactions ye latent complexities in materials.
The careful interpretation of photoluminescence data provides an essential key to unlocking the potential of advanced materials in multiple scientific domains, including nanotechnology and biotechnology.
Thus, the combination of robust measurement techniques and meticulous data analysis allows for a deeper understanding of photoluminescent materials and underpins the innovations that can arise from their study.
Applications in Materials Science
Photoluminescence has carved itself a noteworthy niche in materials science, acting as a cornerstone for various research and technological applications. Understanding photoluminescence can open doors to innovating materials with enhanced properties and capabilities. As industries seek advanced materials for a range of uses, the insights provided by photoluminescence become ever more crucial.
Semiconductor Materials
In the realm of semiconductor materials, photoluminescence serves as a valuable tool for characterizing the optical properties of new compounds. By analyzing the emitted light from a semiconductor when excited by a certain wavelength, researchers can gather essential data that includes bandgap information, defect states, and impurity levels. This is incredibly significant since optimizing these properties is key to developing more efficient electronic devices.
For example, gallium nitride (GaN), a semiconductor commonly employed in LEDs, has its performance deeply influenced by its photoluminescent properties. Specific luminescence signals can indicate the presence of defects or dislocations in the crystal structure of GaN. These imperfections can hinder the device's efficiency, so understanding and mitigating them through photoluminescent analysis can lead to advancements in lighting technology.
Moreover, the exploration of novel materials such as perovskites for solar cells has also benefited from photoluminescence. The light-emitting characteristics of these materials allow researchers to visualize how charge carriers behave, guiding improvements in the energy conversion efficiency. Therefore, the utility of photoluminescence in semiconductor materials cannot be overstated; it is, quite literally, a guiding light for researchers aiming to push the boundaries of technology.
Optoelectronic Devices
When it comes to optoelectronic devices, photoluminescence plays a critical role in developing components like lasers, photodetectors, and sensors. These applications often rely on precise emission characteristics to function effectively. The luminescent behavior of materials can illuminate pathways to enhancing device performance.
Take organic light-emitting diodes (OLEDs) as an example. The efficiency and durability of these devices hinge on understanding the photoluminescence properties of various organic compounds. By tweaking the molecular structure of these compounds, researchers can maximize light emission while minimizing energy loss, thus improving device longevity.
Another area where photoluminescence is pivotal is in the realm of solar energy. Photovoltaic cells depend significantly on the properties of the materials that convert sunlight into electricity. Here, photoluminescence helps in optimizing the light absorption spectrum of the materials, which leads to increased energy harvested from sunlight. In this case, it stands at the crossroads of materials science and energy production, making significant impacts on sustainable technologies.
Through the lens of photoluminescence, materials science not only evolves but pushes the frontiers of innovation, enriching our technological landscape.
In summary, the applications of photoluminescence in materials science are profound. From semiconductor materials to optoelectronic devices, the insights gained help refine and design materials with enhanced optical properties that meet contemporary needs. As the quest for more efficient and effective technologies continues, photoluminescence will undoubtedly remain at the forefront of materials research.
Biological Applications
The interplay between photoluminescence and biology holds enormous potential, carving significant pathways in diagnostics and therapeutics. This section delves into how photoluminescent materials serve as vital tools within biological sciences, offering not only insights into cellular mechanisms but also paving the way for innovative medical practices. The ability to visualize biological interactions in real-time through fluorescent probes is revolutionary, making this field not just relevant but essential for future advancements in medicine and healthcare.
Photoluminescent Probes
In the realm of biological applications, photoluminescent probes are crucial for studying cellular structures and processes. These probes, which exhibit fluorescence, are specifically designed to bind to certain biological targets such as proteins, nucleic acids, or even entire cells. By attaching a photoemissive label, researchers can track cellular activity over time. For instance, when a fluorescent dye binds to a cellular component, it not only illuminates the structure but can also reveal insights into the component's functionality.
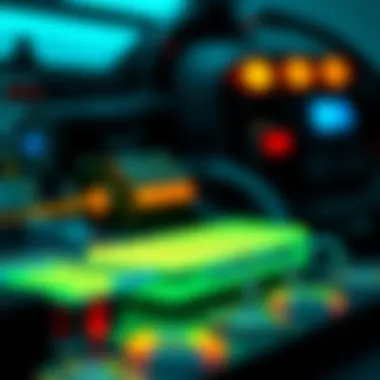
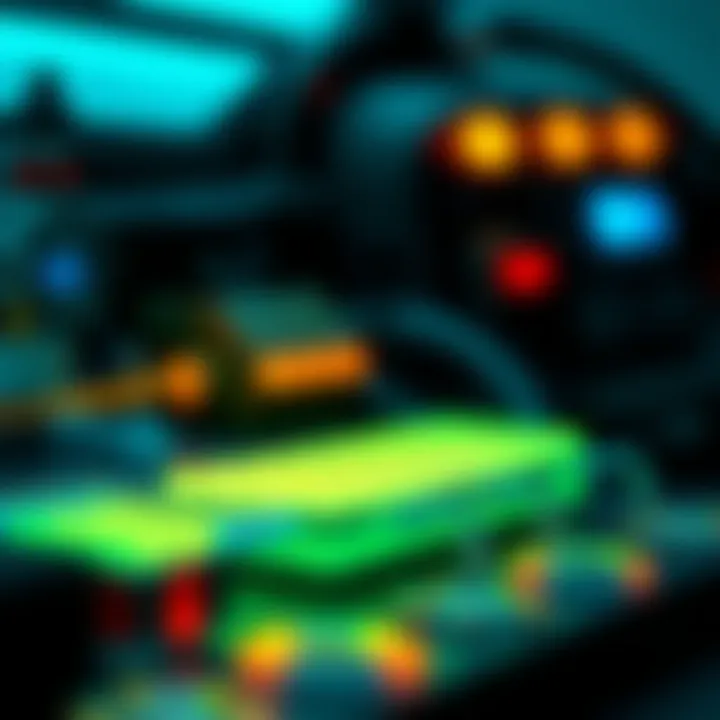
Utilizing various spectral properties, researchers can employ multiple probes simultaneously, providing a multi-faceted view of cellular dynamics. The use of probes like Green Fluorescent Protein (GFP) has significantly enhanced our understanding of cellular interactions by allowing real-time monitoring. This capability is vital for understanding disease mechanisms, drug interactions, and metabolic changes.
- Benefits of Photoluminescent Probes:
- Non-invasive imaging techniques.
- Ability to study live cells over extended periods.
- High specificity due to selective binding properties.
- Real-time data acquisition that improves experimental accuracy.
"Photoluminescent probes have revolutionized biological research by allowing scientists to visualize processes at an unprecedented resolution."
Diagnostic Techniques
The integration of photoluminescence within diagnostic techniques has reshaped traditional methodologies. Various diseases, particularly cancers, have benefited immensely from such advancements, allowing for earlier detection and monitoring. Fluorescence-based assays and imaging methods are becoming staples in clinical laboratories and research institutions worldwide. The techniques harness luminescence for both qualitative and quantitative analysis, providing detailed insights into the biological underpinnings of diseases.
For example, fluorescence in situ hybridization (FISH) allows for the visualization of specific DNA sequences in cells, making it a pivotal technique in genetics and cancer diagnostics. Additionally, photoluminescent markers can reveal the presence of specific proteins or pathogens in a sample, contributing to accurate diagnosis and treatment planning.
- Key Diagnostic Techniques Involving Photoluminescence:
- Fluorescence Microscopy
- Flow Cytometry
- Enzyme-Linked Immunosorbent Assay (ELISA)
Nanotechnology and Photoluminescence
Nanotechnology plays a vital role in the field of photoluminescence, serving as a bridge between fundamental science and practical applications. This intersection is rich with possibilities, paving the way for innovations that could change how we perceive materials and their functionalities. As we dive into this section, it becomes clear that the implications of nanotechnology within photoluminescence extend beyond mere curiosity, entering realms of immense practical and scientific significance.
One of the most fascinating aspects of nanotechnology is its ability to manipulate materials at the atomic and molecular level. This capability results in unique optical properties that can differ vastly from their bulk counterparts. Thus, materials can show enhanced photoluminescent behaviors. Often, when you push materials down to nanoscale dimensions, they exhibit effects like quantum confinement, where the electronic properties, and subsequently the photoluminescence, are fundamentally altered.
Quantum Dots
Quantum dots, tiny semiconductor particles usually ranging between 2 to 10 nanometers in size, are an exemplary illustration of how nanotechnology catalyzes developments in photoluminescence. These nanoscale structures shine brightly because of their size-dependent optical properties. When light strikes a quantum dot, it can absorb photons and re-emit them, resulting in strikingly vivid colors based on the particle’s size. Smaller quantum dots pack a vibrant green, while larger ones glow red.
The versatility of quantum dots extends to their use in various applications, such as in displays or biological tagging. For instance, in biological research, quantum dots can serve as fluorescent tags that help visualize cellular processes in real-time without the extensive harm to the organism being examined. This opens new avenues in research—allowing scientists to track movements and interactions at the cellular level with remarkable precision.
Typically, quantum dots are produced through methods like chemical vapor deposition or colloidal synthesis. However, challenges remain, such as stability and potential toxicity.
Nanocomposites
Following closely behind quantum dots in the nanotechnology scene are nanocomposites, which combine nanomaterials with a matrix of other materials to enhance performance. In the context of photoluminescence, these nanocomposites can inherit unique luminescent properties from their constituents.
For example, incorporating quantum dots into polymer or glass matrices creates nanocomposites that are not only lightweight and durable but also showcase superb photoluminescent characteristics—ideal for applications in lighting and displays. An area of great promise is in creating energy-efficient lighting systems that make use of nanocomposites, significantly pushing the boundaries of what's possible in the lighting industry.
Important to consider when dealing with nanocomposites is the uniformity and stability of the blend. Ensuring that the quantum dots are evenly distributed in a larger material structure can make or break the performance. In essence, this field emphasizes collaboration across various scientific disciplines, intertwining physics, material science, and engineering to unlock new methodologies and enhance existing technologies.
As research advances, the potential for the application of these nanocomposites only grows. Their ability to manipulate light presents vast opportunities in several fields, ranging from consumer electronics to telecommunications.
"The marriage of nanotechnology and photoluminescence not only allows us to create new materials but also to rethink the possibilities within numerous applications across diverse sectors."
In summary, the integration of nanotechnology in photoluminescence serves as a critical focal point for future research and development. Quantum dots and nanocomposites exemplify the groundbreaking advances in material sciences, holding the promise for technology in both industrial and scientific landscapes.
Challenges and Limitations
The realm of photoluminescence, while vibrant and dynamic, is also not without its hurdles. Recognizing the challenges and limitations associated with this phenomenon is crucial for researchers and practitioners who aim for practical implementations and advancements in technology. This section aims to shed light on two key aspects: material stability and efficiency concerns. Both play pivotal roles in determining the viability of photoluminescent materials in various applications.
Material Stability
Material stability refers to the ability of photoluminescent materials to maintain their luminous properties over time, particularly when exposed to environmental factors such as light, temperature, and humidity. When discussing this topic, it's essential to note that photoluminescent materials, especially those employed in devices and system applications, must exhibit stable performance under a range of conditions.
Some materials may initially display remarkable photoluminescent characteristics, only to fade or degrade when exposed to prolonged light, heat, or even chemical interactions. For example, certain dyes used in biological probes can be so sensitive that their efficiency drops significantly just weeks after synthesis. This instability can lead to inaccurate data in experiments and questionable results in clinical diagnostics.
Inconsistent performance can hinder the reliability of photoluminescent materials, forcing researchers to seek alternatives or develop new formulations to enhance stability.
A durable photoluminescent material would ideally show minimal degradation, ensuring consistent data collection and reliable performance in devices. Thus, researchers are investing significant efforts into enhancing the stability of these materials, exploring coatings or composite solutions that can protect luminescent elements from deleterious effects. High-quality materials that resist breakdown under operational conditions greatly extend the lifespan and usefulness of photoluminescent applications.
Efficiency Concerns
Efficiency is another crucial factor, especially when considering the practical utility of photoluminescent systems. In this context, efficiency can refer to several aspects, including the degree of light conversion, energy consumption, and the ratio of useful luminescent output to total energy input. Highly efficient materials are of paramount importance in applications ranging from display technologies to bioimaging.
Take, for example, quantum dots and their application in displays. These nanoscale materials promise extraordinary efficiency, emitting light with minimal energy loss. However, achieving this efficiency consistently across various platforms is still a matter of ongoing research. Factors such as the production method, environmental stability, and compatibility with other components can significantly impact overall efficiency.
Moreover, there are instances where efficiency is traded off for other desirable properties. For instance, a material may offer stunning luminescent properties but at the cost of high energy consumption or a short operational duration. This trade-off can create dilemmas for scientists and engineers in choosing the right materials for their specific applications. An efficient photoluminescent material should not only emit brightly but also sustain its output over longer periods without drawing excessive energy.
By understanding the challenges posed by material stability and efficiency concerns, researchers can tailor their studies and applications towards more robust and dependable photoluminescent materials. This awareness can drive innovations that yield longer-lasting, high-efficiency photoluminescent systems, ultimately enhancing the impact of this field on science and technology.
Recent Advances in Photoluminescent Materials
Recent strides in photoluminescent materials have sparked significant interest among scientists and industry professionals alike. This field is rapidly evolving, pushing the boundaries of how we perceive light-emitting substances. Advances in synthesis techniques and innovative applications are central to these developments. Such progress not only enhances efficiency but also opens doors to new possibilities in technology and medicine.
Synthesis Techniques
Developing novel synthesis methods for photoluminescent materials is crucial in driving innovation. Recent techniques emphasize precision and control over the material characteristics, where factors like particle size, composition, and environment play pivotal roles.
One prominent method is sol-gel synthesis. This approach allows for the creation of homogenous materials at lower temperatures. By manipulating the gel state and transition to solid, researchers can fine-tune the luminescence properties significantly. Another advancement involves hydrothermal synthesis. This method uses high temperatures and pressures to facilitate reactions in a water-based solution, often yielding crystalline structures with reinforced photoluminescent properties. Other exciting avenues include the exploration of biomimetic approaches where natural processes inspire the creation of luminescent materials. This method offers a sustainable angle to material production, potentially reducing the environmental impact.
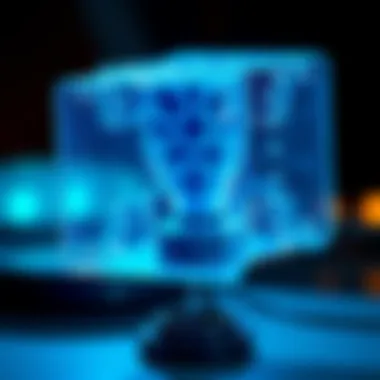
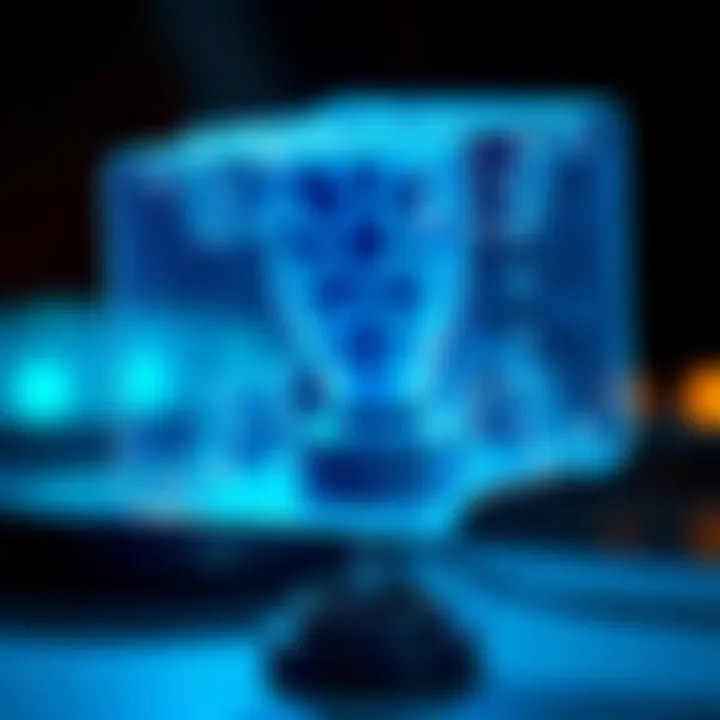
Overall, these synthesis techniques provide a pathway not just to enhance efficiency but also to innovate in terms of applications and functionalities.
Applications in Technology
The implications of these advances stretch across various domains, impacting areas such as optical devices, sensing, and energy harvesting. As technology demands greater efficiency and performance, photoluminescent materials are integrating into systems that require exceptional light interaction.
In display technologies, organic light-emitting diodes (OLEDs) are a perfect case in point. Recent developments have led to materials that emit brighter, longer-lasting light while consuming less power. These enhancements translate into vivid imagery and reduced energy costs, a win-win for manufacturers and consumers alike.
Additionally, in the realm of sensing, photoluminescent materials are being utilized in environmental monitoring and biochemical assays. They enable highly sensitive detection methods that respond to minute changes in their surroundings. For example, luminescent probes are capable of signaling the presence of specific ions or molecules in real-time, providing crucial data across health sciences and environmental studies.
Furthermore, as renewable energy gains traction, photoluminescent materials are finding their way into solar cells. By increasing absorption and reducing energy loss, these materials enhance the efficiency of energy conversion processes.
The intersection of innovation in synthesis and application development positions photoluminescent materials at the forefront of technological advancement, making their study both timely and crucial for future progress.
In summary, recent advances in both synthesis techniques and applications highlight the dynamic potential of photoluminescent materials. These materials are not merely passive components; they’re active agents of change in science and technology, offering improved performance, sustainability, and unique functionalities.
Future Trends and Directions
Understanding the future trends and directions in photoluminescence is crucial for both scientific inquiry and practical applications. As technology evolves, so too do the methods of harnessing light interactions with materials. This section recognizes significant pathways where photoluminescence can impact a variety of fields, from information technology to material science and medicine.
Integration with Emerging Technologies
With the rapid pace of innovation in technology, integrating photoluminescence with emerging methods such as artificial intelligence, machine learning, and nanotechnology is highly promising. These areas can amplify the applications and functionality of photoluminescent materials. For instance, artificial intelligence enhances the efficiency of material discovery, optimizing conditions for synthesis through predictive modeling.
Consider the potential of using machine learning algorithms to predict how different photoluminescent compounds will behave under various conditions. This not only streamlines the experimental process but also reduces the time and resources spent on trial and error. Here are some noteworthy integrations:
- Advanced Imaging Techniques: Photoluminescent materials can be combined with high-resolution imaging technologies, making the visualization of complex biological processes more effective.
- Smart Packaging: Incorporating photoluminescent markers in packaging can help in tracking product freshness, decreasing waste in industries like food and pharmaceuticals.
- Data Communications: The potential for photonic devices that utilize photoluminescence for data transmission is worth noting. This could revolutionize how we approach data networks, enhancing speed and bandwidth.
"Interdisciplinary collaborations are often where real breakthroughs occur, pushing the boundaries of what's possible in photoluminescent applications."
Potential for Medical Innovations
Photoluminescence holds remarkable potential for medical advancements. In diagnostics and therapeutics, employing photoluminescent probes can lead to early disease detection and targeted treatments.
In diagnostics, certain photoluminescent compounds can be tailored to respond to specific biological markers, enabling researchers to visualize anomalies at the cellular level. This specificity enhances accuracy in identifying conditions, such as cancers, with greater efficiency than traditional methods.
- Imaging Technologies: Photoluminescent markers are invaluable in imaging modalities. Techniques like fluorescence in situ hybridization (FISH) rely on photoluminescence to highlight specific genetic material within cells, facilitating genetic analysis.
- Targeted Therapy: Photoluminescent nanoparticles can be engineered to target and treat tumors, reducing side effects commonly associated with conventional treatments. By attaching drugs to these particles, they can be activated only at the tumor site, leading to more effective treatments.
- In Vivo Monitoring: Methods employing photoluminescent probes for real-time tracking of cellular processes represent a qualitative leap in understanding physiological conditions.
As these modalities advance, the role of photoluminescence in medical technology will become more pronounced, bridging gaps between line-of-sight applications and groundbreaking discoveries.
Interdisciplinary Perspectives
The notion of interdisciplinary perspectives is paramount when exploring the complexities of photoluminescence. This area of study seamlessly interweaves multiple scientific domains, presenting a mosaic of insights that enhance the overall understanding and practical application of photoluminescent materials. By incorporating principles from physics, chemistry, biology, and material science, researchers unlock novel pathways, leading to innovative solutions to real-world problems and paving the way for future technological breakthroughs.
A critical aspect of this interdisciplinary approach is the collaboration between disciplines. When experts from varied backgrounds band together, they merge their respective strengths, allowing for a richer exploration of photoluminescence. For instance, physicists who comprehend the fundamental mechanisms can work alongside chemists proficient in synthesizing luminescent materials. Such synergies can result in advanced photonic devices and sensors with unprecedented performance.
Moreover, this cross-pollination of ideas fosters creativity and encourages out-of-the-box thinking, helping to tackle challenges that no single discipline could overcome alone. The sharing of knowledge leads to insights regarding the scaling of laboratory successes to full-scale applications in industry, allowing practical benefits to come forth rapidly.
Collaboration between Disciplines
When discussing collaboration, one must consider the direct benefits that arise from interdisciplinary teamwork. For example:
- Enhanced Innovation: Combining knowledge from physics, chemistry, and engineering cultivates groundbreaking applications in imaging technology and biomedical diagnostics.
- Comprehensive Problem Solving: By pooling expertise, interdisciplinary teams can address complex challenges in photoluminescence, such as improving material stability or optimizing light emission efficiency.
- Tailored Applications: Researchers can develop specific solutions for various fields; for instance, designing specialized photoluminescent probes for biological applications that are fine-tuned for certain target cells.
Guided by a shared vision, multidisciplinary collaborations can harness diverse viewpoints and skill sets, ultimately leading to robust advancements in photoluminescent technologies.
Impact on Scientific Research
The ripple effect of interdisciplinary work in photoluminescence extends deeply into scientific research. Each collaboration not only augments individual studies but also propels the broader field forward. Here are a few key impacts:
- Accelerated Progress in Understanding: Integrating insights from various scientific traditions can significantly shorten the timelines for breakthroughs in understanding photoluminescence.
- Increased Funding Opportunities: Projects that bridge multiple disciplines often attract more funding as they address wider applications and implications, making a compelling case for resources.
- Boost in Publishing and Recognition: Interdisciplinary research often garners attention across multiple academic fields, leading to higher publication rates and greater recognition for contributors.
"Advance in one discipline can ignite progress in another, catalyzing a cascade of innovation that benefits society as a whole."
As technology continues to evolve, the interconnections between differing fields will only become more pronounced, enhancing both the theoretical and applied aspects of photoluminescence research. The interdisciplinary perspectives not only enrich scientific inquiry but also lay down the groundwork for future advancements, potentially leading to breakthroughs that transform our approach to both science and technology.
Closure
The conclusion of our exploration into photoluminescence draws together a myriad of threads that underline its significance across multiple disciplines. Understanding photoluminescence is not just an academic exercise; it has practical implications that can resonate in everyday applications. By synthesizing the fundamental mechanisms, types, and challenges faced in the use of photoluminescent materials, we gain valuable insights into how light and matter interact. Such perspectives are particularly relevant in fields like materials science, biotechnology, and even nanotechnology.
Summation of Key Points
In summarizing the key points discussed throughout the article, the interplay between excitation and emission processes remains central to understanding photoluminescence.
- Definition: Photoluminescence, essentially, is the light emitted after a material absorbs photons.
- Mechanisms: At the core, excitation and emission processes shape the photoluminescent behavior.
- Types: The distinction between fluorescence and phosphorescence is critical, highlighting different duration and energy release.
- Applications: We’ve seen how this phenomenon is harnessed in a variety of contexts, from semiconductors to diagnostic tools in medicine.
- Challenges: Factors like material stability and efficiency are hurdles that researchers continue to address.
"Knowledge is power, and understanding photoluminescence is key to unlocking new technologies and innovative solutions across diverse fields."
Final Thoughts
In reflecting upon the future prospects of photoluminescent materials, it becomes clear that the continued advancements could lead to remarkable breakthroughs in technology and science. The integration of photoluminescence with emerging fields, such as quantum computing and renewable energy, presents a landscape ripe for exploration.
Moreover, the interdisciplinary collaboration highlighted in our discussion emphasizes that true innovation often occurs at the boundaries between distinct research domains. As researchers, students, and practitioners continue to explore and innovate, the potential applications of photoluminescence seem virtually limitless.
Thus, for the inquisitive mind, delving deeper into the nuances of photoluminescence is both imperative and exciting. A thorough grasp of this phenomenon can inspire further research efforts that not only advance technology but also enhance our understanding of the fundamental nature of light and matter.
Embracing this knowledge ultimately leads to greater innovations that can significantly benefit society.