Hydrogen Production through Water Electrolysis
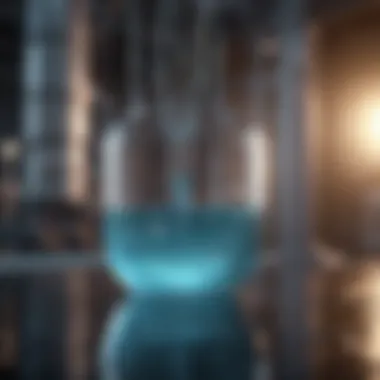
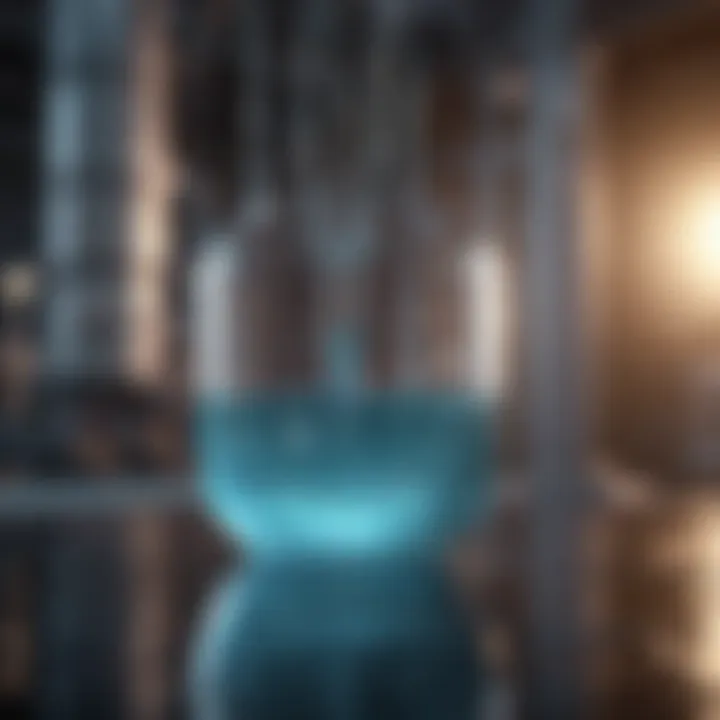
Intro
Producing hydrogen through water electrolysis is a critical subject that sits at the crossroads of energy sustainability and technological innovation. Over recent years, there has been a burgeoning interest in hydrogen as a clean energy carrier. It can potentially replace fossil fuels and contribute significantly to a greener future. This article dives into the diverse methodologies of hydrogen production via electrolysis and explores their implications for both science and industry.
The essence of electrolysis lies in the fundamental process of splitting water into hydrogen and oxygen using electrical energy. This method simplifies the understanding of hydrogen production by creating a cleaner solution with minimal carbon footprint compared to traditional methods, like steam methane reforming.
Overview of Research Topic
Brief Background and Context
Water electrolysis, though a relatively straightforward process, showcases significant potential in the modern energy landscape. The roots of electrolysis can be traced back over two centuries, to the early experiments of scientists such as William Nicholson and Anthony Carlisle. Their pioneering work laid the groundwork for what we recognize today as a pivotal tool in sustainable energy.
With growing concerns around climate change and increasing energy demands, the significance of hydrogen production has surged. Innovative advancements have integrated renewable energy sources like wind and solar into the electrolysis process, making this method not just viable but a cornerstone of future energy systems.
Importance in Current Scientific Landscape
Hydrogen is often branded as the fuel for the future—its versatility transcends transportation and extends to industrial applications and electrical generation. The role of hydrogen in energy systems is compelling, primarily as countries aim to meet ambitious greenhouse gas reduction goals.
With the global energy sector entering a transitional phase, pursuing clean hydrogen production through water electrolysis addresses several key concerns:
- Sustainability: As electrolysis uses abundant water and renewable energy, it's an environmentally friendlier alternative.
- Versatility: Hydrogen can be deployed across various sectors from transport to industrial processes.
- Storage: Hydrogen provides a convenient method for storing excess renewable energy, supporting grid stability.
Nonetheless, the journey toward a hydrogen economy is not without its hurdles. Technical challenges, market adoption rates, and the development of necessary infrastructure need to be navigated effectively to unlock the full potential of this clean energy source.
"The future of hydrogen is not just about production; it's about embracing a new energy paradigm that prioritizes sustainability and practicality."
Methodology
Research Design and Approach
In crafting this exploration, a qualitative approach has been employed to dissect the various aspects of hydrogen production through electrolysis. This methodology emphasizes a comprehensive understanding of the technological, economic, and environmental facets involved.
Data Collection Techniques
- Literature Review: In-depth analysis of current studies and publications on hydrogen production, focusing on case studies and technological advancements.
- Interviews: Engaging with experts and industry stakeholders to gain insights into the market dynamics and challenges faced in the field.
- Industry Reports: Reviewing data from influential organizations to gather empirical evidence on current progress and future trends in hydrogen production.
By synthesizing findings from these methods, the narrative aims to provide a thorough examination of the landscape surrounding hydrogen production and its implications for the transition to sustainable energy.
Understanding Water Electrolysis
Water electrolysis serves as a cornerstone in hydrogen production and the broader conversation surrounding sustainable energy sources. To grasp its significance, it is essential to first break down what water electrolysis entails—an electrochemical process that disassociates water into its fundamental elements: hydrogen and oxygen. This process doesn’t just offer a means of hydrogen generation; it also plays a pivotal role in enhancing the viability of renewable energy systems, bridging the gap between fluctuating energy supply and demand.
The importance of this topic extends beyond the mere mechanics of electrolysis. Understanding the mechanisms behind it allows for optimization in hydrogen output, effective energy use, and the integration of variable renewable energies like solar and wind. Water electrolysis not only has the potential to revolutionize hydrogen production but also contributes to resolving broader energy concerns by promoting the use of clean technologies.
Moreover, as nations buckle down on carbon neutrality goals, water electrolysis is increasingly viewed as not just an option, but a necessity. It embodies the shift toward greener practices, reducing reliance on fossil fuels. Thus, understanding these fundamental principles is not a mere academic exercise—it is a necessity for anyone looking to grasp the future landscape of energy solutions.
The Basics of Electrolysis
Definition of Electrolysis
Electrolysis is fundamentally the splitting of water into hydrogen and oxygen through the application of an electrical current. The simplicity of this definition belies the intricate physical and chemical principles underlying the process. One of its key characteristics is that it can be powered by renewable energy sources, making it a popular choice for producing clean hydrogen.
A notable aspect of electrolysis is its efficiency—it can yield high-purity hydrogen, crucial for various applications. However, the initial energy requirements to drive the process can sometimes be high, raising questions about its overall energy efficiency in comparative scenarios against other hydrogen production methods. Therefore, these considerations form the crux of ongoing research efforts aimed at enhancing the overall effectiveness of electrolysis.
Key Components of an Electrolytic Cell
Diving deeper into the shoals of water electrolysis, an electrolytic cell comprises three core components: the anode, cathode, and the electrolyte. Each part plays a vital role in facilitating the reactions that lead to hydrogen production. The anode, where oxygen is generated, and the cathode, the site of hydrogen generation, function in tandem to harness electrical energy effectively.
The selection of materials for these components is crucial. Materials need to be conductive while also resilient to corrosion—after all, the environment within the cell is harsh. When selecting appropriate materials, one must balance cost with performance characteristics, making this a prime focus for ongoing innovation in this field.
Electrolyte Selection
The chosen electrolyte significantly impacts the efficiency of water electrolysis. Generally, an electrolyte must favorably conduct ions while providing a conducive environment for chemical reactions. Commonly used electrolytes include potassium hydroxide and phosphoric acid due to their conductive properties and stability under operational conditions.
A unique feature of electrolyte selection arises when considering temperature ranges and conductivity, as these can vastly change the efficiency of the electrolysis process. Choosing an appropriate electrolyte isn't just a technical decision; it holds implications regarding cost and operational longevity. Thus, it’s a pivotal aspect that’s well worth keeping an eye on as technology progresses.
Chemical Reactions in Electrolysis
Oxygen Evolution Reaction
At the heart of water electrolysis lies the oxygen evolution reaction (OER). This process involves transporting electrons from water molecules to create oxygen gas and protons, consequently affecting the overall efficiency and energy requirements of the system. The OER can have a significant potential impact on the efficiency of the entire electrolysis process, as it is often more energy-intensive than the opposing reactions.
What makes OER particularly fascinating is its complexity—a myriad of factors, including pH levels and the choice of catalyst materials, can affect how efficiently this reaction occurs. It stands out as a significant subject for researchers who aim to enhance performance and reduce energy costs.
Hydrogen Evolution Reaction
Complementing the OER is the hydrogen evolution reaction (HER), which is where hydrogen gas is generated at the cathode. The HER is generally considered less complicated compared to OER, however, it still embodies its challenges.
Key characteristics informing the HER involve selecting appropriate catalysts that can lower activation energy. Notably, the HER not only serves as the endpoint for hydrogen production—it can also influence ongoing operational costs as the efficiency of this reaction directly correlates to the overall productivity of the system. Thus, detailed attention to the nuances of the HER is indispensable.
Overall Reaction in Water Electrolysis
When looking at the overall reaction in water electrolysis, it can be distilled down to a simple yet profound equation: 2H₂O → 2H₂ + O₂. This reaction encapsulates the heart of this process and highlights its potential to yield hydrogen as a clean energy carrier. The straightforward nature of this reaction can be misleading; understanding the underlying processes allows for optimization in operational parameters.
The beauty of this overall reaction lays not just in its simplicity, but also in the potential impact—clean hydrogen can be utilized across various sectors, thus fostering the transition towards sustainable practices. This synthesis underscores the essence of water electrolysis and its role in addressing some of the most pressing energy challenges today.
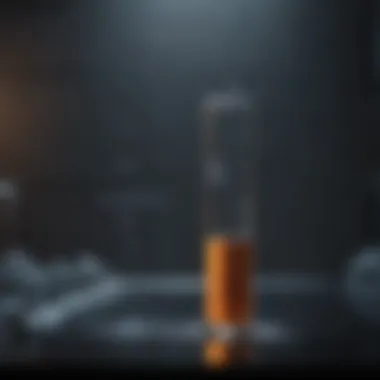
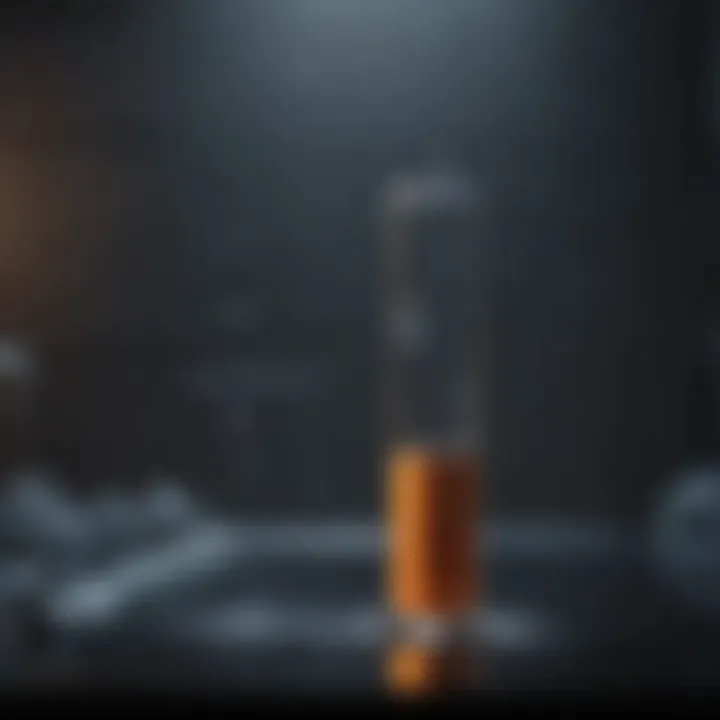
"Water electrolysis is more than just science; it's a gateway to a sustainable future, re-engineering how we view energy sources."
In summary, a thorough understanding of water electrolysis—its definition, core components, chemical reactions, and their intricacies—provides the foundational knowledge necessary for unlocking the broader potentials of hydrogen production. As we peel back the layers of this process, we pave the way toward effective implementations that promise not only advancements in technology but also a profound impact on energy sustainability.
Types of Electrolysis Techniques
The production of hydrogen through water electrolysis involves several techniques, each with unique mechanisms, efficiencies, and areas of application. Understanding the different types of electrolysis methods is pivotal for identifying the best approach to harnessing hydrogen's potential as a clean energy source. This section will explore the various electrolysis techniques that are currently in use, highlighting their characteristics, advantages, and limitations.
Alkaline Electrolysis
Mechanism and Applications
Alkaline electrolysis involves the use of a liquid alkaline electrolyte, commonly potassium hydroxide, to facilitate the reactions that produce hydrogen and oxygen. The basic mechanism is simple: an electric current is passed through the electrolyte, allowing the water molecules to split into hydrogen ions and hydroxide ions. The process predominantly occurs at the electrodes, where it effectively generates hydrogen gas at the cathode and oxygen gas at the anode.
One key characteristic of alkaline electrolysis is its relatively low operational costs compared to other techniques. Many industries have adopted it primarily due to its scalability. Additionally, its ability to utilize various types of renewable energy sources makes it a popular choice for large-scale hydrogen production. Its unique feature lies in the capacity for robust production systems, which can significantly contribute to hydrogen economies.
However, the system doesn’t come without disadvantages. The type of materials used in electrodes can limit efficiency; for instance, they may degrade faster in alkaline conditions, impacting the longevity of the system.
Advantages and Disadvantages
When examining the advantages, alkaline electrolysis shows a relatively high Faradaic efficiency, mostly due to the substantial driving force provided by the alkaline electrolyte. Furthermore, these systems tend to have a lower initial investment compared to other electrolysis methods, making them suitable options for startups looking to enter the hydrogen market.
On the flip side, the limitations include a slower response time to changes in power input – which can be detrimental in applications where quick adaptation is necessary – and a larger footprint relative to more compact systems like PEM electrolyzers. This might limit the application in space-constrained environments, where efficiency in both production and space usage is necessary.
Proton Exchange Membrane (PEM) Electrolysis
Operational Principles
In contrast to alkaline methods, PEM electrolysis employs a solid polymer electrolyte membrane to conduct protons while isolating reactants at the electrodes. When a voltage is applied, water molecules are ionized at the anode, and the positively charged protons move through the membrane to the cathode, where they combine to form hydrogen gas.
The key characteristic of this method is its ability to operate at a high range of pressures and temperatures, lending itself to various industrial applications. Its operational flexibility mainly speaks to its compatibility with power input from renewable energy sources, which varies erratically. Consequently, PEM electrolysis can become an especially attractive option for hydrogen production in conjunction with wind and solar power systems.
Nevertheless, the technology is not without its challenges. The cost of PEM systems, particularly due to the precious metals used in the electrodes, can present significant economic barriers.
Potential Uses and Limitations
PEM electrolysis exhibits a myriad of potential uses, especially in energy storage systems combined with renewable energy generation. By stabilizing energy output from renewable sources, these systems can alleviate variability challenges commonly encountered in energy systems reliant on solar or wind power. The unique feature of PEM electrolyzers is their rapid startup time, allowing them to come online quickly to match supply and demand fluctuations.
However, these advantages come with limitations. The high cost of materials needed for construction and the maintenance of PEM systems can be a deterrent for mass deployment. Additionally, achieving optimal performance usually requires careful control over operational conditions, adding further complexities to the overall setup.
Solid Oxide Electrolysis
High-Temperature Electrolysis
Solid oxide electrolysis operates at elevated temperatures, often exceeding 600°C. The high-temperature environment enables more efficient electrolysis compared to lower temperature methods, significantly reducing the energy required for hydrogen production. The solid oxide electrolyte separates reactions occurring at the anode and cathode, allowing for effective ion transport while minimizing energy loss.
This method holds immense potential due to its efficiency. One standout characteristic is its ability to directly utilize steam instead of liquid water, enhancing energy efficiency even more. The investments in high-temperature technologies have been encouraged due to the long-term viability of hydrogen production.
Despite its benefits, high-temperature electrolysis also has drawbacks. The need for advanced materials that can withstand severe conditions presents a challenge, alongside the complexity of system design and integration into existing infrastructures.
Prospects for Industrial Applications
Solid oxide electrolysis has promising industrial applications, ranging from energy production to synthesis of fuels and chemicals. The method can effectively convert excess heat from industrial processes into hydrogen, providing a dual benefit of waste heat recovery and clean hydrogen generation. The unique feature of incorporating captured CO₂ in these processes makes it particularly attractive for sectors looking to reduce their carbon footprint.
Nonetheless, high installation and operational costs can deter widespread adoption. Industries looking for immediate cost-effectiveness may not favor it, despite the long-term returns on investment that high-temperature solutions offer.
Microbial Electrolysis
Biological Processes Involved
Microbial electrolysis relies on the metabolic processes of microorganisms to facilitate electrolysis. This approach leverages the ingenious ability of specific bacteria to produce hydrogen gas during the breakdown of organic materials under an electric field. The key characteristic of microbial electrolysis lies in its ability to utilize waste organic material, promoting a circular economy approach to hydrogen production.
Specific features include its potential for carbon-negative hydrogen production, which can play an essential role in mitigating climate change. It offers a unique pathway for integrating waste management practices with energy generation, turning organic waste into a valuable resource.
However, the complexities associated with maintaining optimal microbial populations and the slower rates of hydrogen production compared to traditional techniques are notable disadvantages.
Alternative Applications
The unique aspect of microbial electrolysis is its expanded usage beyond traditional energy applications. For instance, the technology can be applied in remote locations lacking reliable electricity sources, encouraging decentralized hydrogen generation. Furthermore, by integrating this method with wastewater treatment facilities, cities can produce hydrogen while simultaneously addressing waste disposal.
In terms of drawbacks, the process is often sensitive to environmental changes, which can impact the performance. This type of electrolysis may not yield the large-scale hydrogen outputs typically required for industrial applications, posing challenges for wide-scaled adoption.
Through these layers of understanding in different electrolysis techniques, it becomes clear that each method has its place in the overarching quest for sustainable hydrogen production. The importance of choosing the right technique can't be overstated, as it significantly impacts economic viability, efficiency, and environmental considerations as hydrogen production systems evolve.
Efficiency and Performance Metrics
When delving into hydrogen production via water electrolysis, the concepts of efficiency and performance metrics emerge as cornerstones of any discussion. These metrics not only play a crucial role in determining how effectively hydrogen can be generated, but they also inform research and development efforts aimed at optimizing production methods. Efficiency in electrolysis can signify the difference between a sustainable energy solution and an untenable one, with implications that stretch from economic viability to environmental sustainability. The focus here will be on energy efficiency as well as Faradaic efficiency, both pivotal in assessing the performance of electrolytic processes and with far-reaching consequences on the hydrogen economy.
Energy Efficiency of Electrolysis
Just as a car's fuel efficiency plays a vital role in determining its practicality, the energy efficiency of electrolysis dictates how much energy is consumed in the hydrogen production process relative to the amount produced. \n
Voltage and Current Considerations
Voltage and current stand out as critical elements in assessing the energy efficiency of electrolysis. A well-optimized voltage ensures that the required potential is activated for the electrolysis reaction to proceed without excessive energy waste. Higher voltage demands can lead to increased power consumption without corresponding gains in hydrogen yield.
- Key Characteristic: The relationship between voltage and current sets the foundation for energy consumption. While higher voltage might speed up reactions, excessively increasing it might promote side reactions that lead to losses in effectiveness.
- Unique Feature: The careful balance of voltage and current allows operators to modulate the efficiency of the process. By selecting the right voltage, it's possible to maintain an optimal current that provides consistent hydrogen production without overtaxing the system.
- Advantages/Disadvantages: One of the main benefits of focusing on voltage and current optimization is the possibility of decreasing electricity costs, which represent a significant portion of electrolysis expenses. However, overly aggressive current settings might harm the longevity of system components.
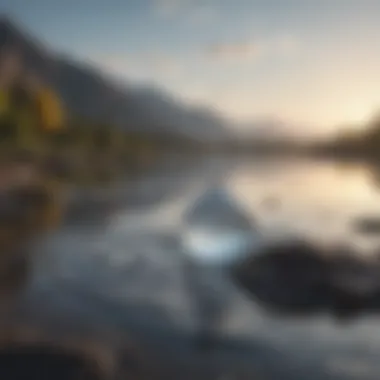
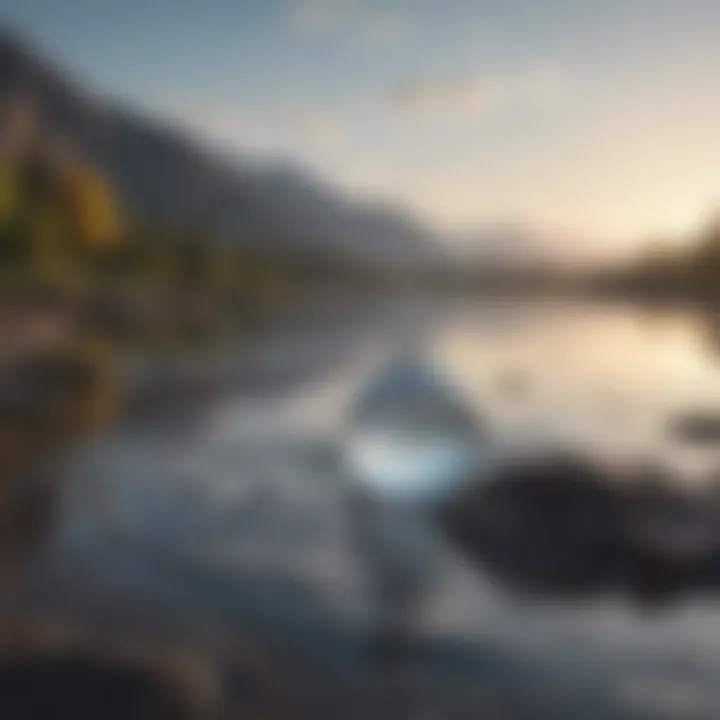
Electrode Material Influence
The choice of electrode material is pivotal when it comes to the performance of electrolysis systems. Different materials can exhibit varying rates of hydrogen evolution and resistance to corrosion, crucial factors in determining how efficiently water can be split into hydrogen and oxygen.
- Key Characteristic: The selection of materials such as platinum, iridium, or nickel can dramatically influence overall efficiency and cost-effectiveness in the long term.
- Unique Feature: Certain materials can enhance the electrode performance by facilitating the separation of ions, promoting faster reactions and minimizing energy losses.
- Advantages/Disadvantages: While premium materials like platinum significantly increase efficiency, their high costs present financial hurdles. Alternative materials may reduce costs, but often at the expense of overall performance longevity and efficiency.
Faradaic Efficiency
Faradaic efficiency is another critical metric in water electrolysis. It provides a measure of the extent to which the electric charge supplied to an electrolytic cell results in the desired chemical reaction, specifically how much hydrogen is generated per unit of charge. A high Faradaic efficiency suggests that most of the input energy is effectively transformed into usable hydrogen, making it a valuable measure of overall cell efficiency.
Determining Hydrogen Yield
Determining hydrogen yield underpins the entire evaluation of electrolysis performance. The measurement focuses on the quantity of hydrogen produced relative to the theoretical maximum based on the input current.
- Key Characteristic: Accurate determination allows for an assessment of how efficiently the process converts electrical energy into chemical energy stored in hydrogen.
- Unique Feature: By accurately measuring the yield, researchers can fine-tune operational parameters to enhance overall system efficiency.
- Advantages/Disadvantages: Higher hydrogen yield translates directly into economic viability; however, achieving such yields often demands careful balance in reaction conditions, which may not always be manageable under real-world scenarios.
Impact of Reaction Conditions
The reaction conditions in an electrolytic cell significantly sway the outcomes of hydrogen production. Factors including temperature, pH, and concentration of electrolyte solutions can all affect performance metrics.
- Key Characteristic: Understanding how these conditions interact informs both efficiency improvements and production costs, allowing for strategic adjustments.
- Unique Feature: Control over these parameters can lead to varying efficiencies; for instance, raising the temperature can lower the energy requirement for the overall process.
- Advantages/Disadvantages: Optimizing reaction conditions is beneficial as it can lead to higher efficiencies, yet they may prove difficult to manage in large-scale operations, requiring ongoing adjustments and monitoring.
The discussion surrounding efficiency metrics encapsulates the essence of advancing electrolysis technologies and their applications in a sustainable hydrogen economy. By honing in on energy usage and output, researchers and industry professionals can unlock significant improvements in hydrogen production.
Applications of Hydrogen from Electrolysis
The applications of hydrogen produced through electrolysis are a cornerstone in the pursuit of sustainable energy solutions. As societies aim to reduce carbon emissions and shift towards cleaner alternatives, hydrogen presents itself as a versatile energy carrier. This section uncovers how hydrogen can be harnessed across various sectors, highlighting specific elements, benefits, and considerations.
Fuel Cells
Working Principles of Fuel Cells
Fuel cells operate by converting the chemical energy from hydrogen into electrical energy. This process relies on an electrochemical reaction where hydrogen molecules are split into protons and electrons. The electrons flow through an external circuit, creating electricity. The unique aspect of fuel cells is their ability to produce energy continuously as long as there is a supply of hydrogen and oxygen. This characteristic makes them an efficient choice since they generate power without combustion, hence avoiding harmful emissions. One of the main benefits of fuel cells is their efficiency, sometimes exceeding that of traditional combustion engines. However, a challenge remains in the production and supply of hydrogen at scale, impacting their widespread adoption.
Integration with Hydrogen Production
Integrating hydrogen production with fuel cell technology creates a symbiotic relationship that enhances energy efficiency. A defining feature of this integration is the model in which renewable energy sources, such as wind or solar, produce hydrogen via electrolysis, which can then be utilized in fuel cells. This method not only stabilizes the energy supply but also promotes the use of green hydrogen, vastly beneficial from an environmental perspective. Nevertheless, the infrastructure required for widespread hydrogen deployment remains a hurdle, making it a less attractive option compared to more established forms of energy.
Industrial Processes
Hydrogen in Ammonia Production
The production of ammonia is one of the most significant uses of hydrogen, particularly in agriculture as a key ingredient in fertilizers. Hydrogen acts as a building block for ammonia synthesis, most commonly through the Haber process. This ties directly into the notion of hydrogen's importance since the fertilizers produced are vital for global food production. A particularly noteworthy feature of this process is that it can potentially shift from fossil fuel-derived hydrogen to green hydrogen sourced from electrolysis, which could drastically cut emissions. However, the transition to using green hydrogen has its challenges, particularly concerning cost and scalability of production.
Role in Steel Manufacturing
In the steel industry, hydrogen is emerging as an alternative to carbon-intensive methods for iron reduction. Utilizing hydrogen instead of coke in this process can significantly reduce CO2 emissions. The pressing characteristic of this approach is its capacity to meet stringent environmental regulations while maintaining productivity. Employing hydrogen in steel production can transform the industry, but the transition will require substantial investments in technology and infrastructure to be effective on a large scale.
Energy Storage Solutions
Hydrogen as a Storage Medium
One of the intriguing aspects of hydrogen lies in its role as an energy storage medium. It can be generated during periods of excess electricity production from renewable sources and stored for later use, effectively acting as a battery for hydrogen energy. This is especially critical when fluctuating energy output from renewable sources like solar and wind is considered. A key advantage of hydrogen as a storage medium is its high energy density compared to conventional batteries, allowing for long-term energy storage. The main downside, however, is the costs associated with hydrogen storage solutions, both in terms of production and the necessary infrastructure to store it safely and efficiently.
Comparison with Battery Technologies
Hydrogen storage systems often get compared to battery technologies in energy applications. While batteries are generally effective for short-term storage, they struggle with long-lasting energy supply. Hydrogen systems, on the other hand, provide a more stable and longer-lasting energy solution. A notable feature of hydrogen storage is its scalability; as demand increases, hydrogen systems can expand to larger scales more readily than traditional battery systems. But, like all technologies, hydrogen storage is not without its drawbacks. The current issues around hydrogen production cost and efficiency can limit its attractiveness compared to established battery solutions, which have seen significant advancements in recent years.
The potential of hydrogen from electrolysis is vast, encompassing a spectrum of applications that can significantly impact the global energy landscape and promote cleaner technologies across industries.
Environmental Considerations
Understanding the environmental considerations surrounding hydrogen production through electrolysis is paramount. As the global community shifts toward sustainable energy models, it becomes crucial to analyze how hydrogen production impacts the environment. Evaluating the carbon footprint and water consumption not only informs policy decisions but also shapes public perception and acceptance of this technology.
Carbon Footprint Analysis
Renewable Energy Sources for Electrolysis
Using renewable energy sources for electrolysis stands out as a vital facet of reducing overall carbon emissions. These sources, such as solar, wind, and hydroelectric power, provide a cleaner electricity supply that can significantly lessen the environmental burden of hydrogen production. By opting for renewables, the key characteristic is their ability to minimize lifecycle emissions, making it a popular choice in the green energy narrative.
In particular, the integration of solar panels has grown in urban and rural settings alike. Many facilities employ them to produce hydrogen, achieving a unique synergy between energy generation and electrolysis. The advantage of this approach lies in the potential for localized hydrogen production, reducing transmission losses and associated emissions.
However, challenges persist. The intermittency of renewable sources means that production can be sporadic. Systems must be designed to balance production with consumption reliably. It requires a robust grid infrastructure that can accommodate variable energy supplies.
Comparative Lifecycle Emissions
The analysis of Comparative Lifecycle Emissions plays a pivotal role in determining the environmental viability of hydrogen production. This concept assesses the total emissions produced at every stage, from resource extraction to the end use of hydrogen.
What substantiates the benefit of this analysis is that it provides a holistic view rather than a narrow focus on immediate emissions. For example, hydrogen derived from fossil fuels explores its hidden costs related to carbon outputs across its lifecycle. In contrast, renewably-generated hydrogen exhibits a starkly different profile, often culminating in lower end-use emissions, especially if society moves toward a zero-emissions goal.
The downside of lifecycle assessment is the complexity involved. It requires extensive data collection and modelling, which can be resource-intensive. Misinterpretation of data can lead to misguided policies based on an incomplete picture.
Water Use and Management
Another essential concern is water use and management in hydrogen production. Electrolysis requires substantial water resources, prompting a need for sustainable sourcing practices.
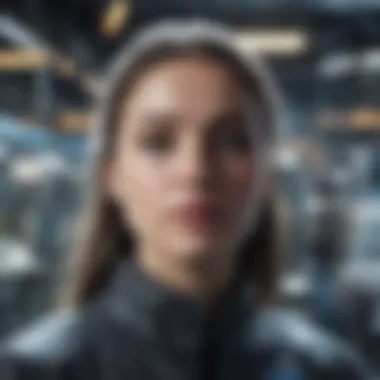
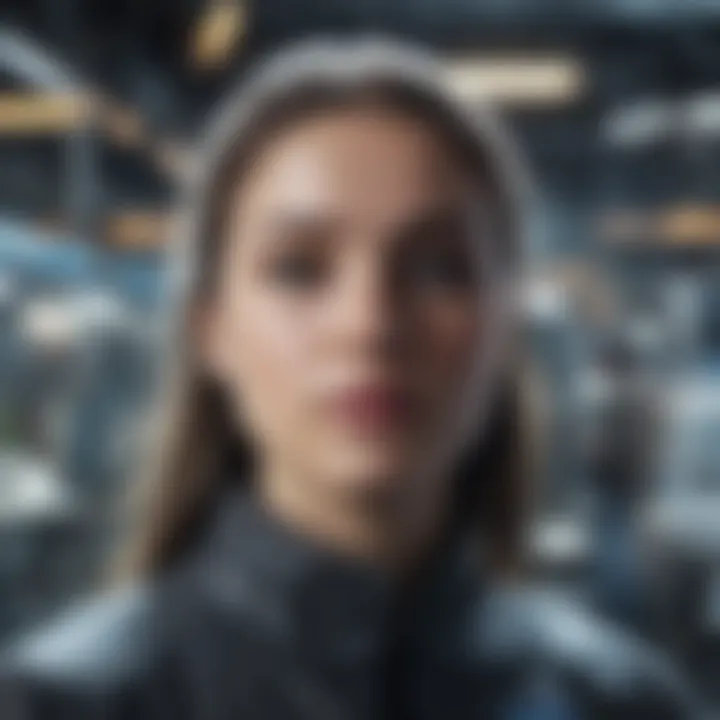
Sourcing Water Sustainably
The importance of sourcing water sustainably cannot be overstated. The focus here is not merely on securing enough water for operations but on ensuring that such practices do not lead to depletion or degradation of local water sources.
One beneficial aspect of adopting sustainable sourcing methods is diversification. Using rainwater harvesting and recycled wastewater systems becomes a viable approach to minimize the negative environmental impacts inherent in electrolysis. These methods also lend themselves to creating a community-focused approach, where water becomes part of a broader ecological management strategy.
Still, the challenge lies in scalability. As hydrogen production ramps up, ensuring that sustainable sourcing matches increased demand is crucial. Otherwise, facilities might unintentionally revert to less sustainable practices, potentially locking in harmful habits.
Impact on Local Water Resources
Finally, the Impact on Local Water Resources must be meticulously evaluated. The withdrawal of water for electrolysis can strain local ecosystems, primarily if used in areas already experiencing water scarcity.
This feature underscores the need for comprehensive planning when installing new hydrogen production facilities. Benefits include ensuring that any water use does not disrupt agricultural practices or the local aquatic habitats. Recently, approaches integrating water impact assessments into the planning phase stand to mitigate adverse outcomes. However, the downside arises when these assessments are overlooked, leading to conflicts over water access and quality.
"Effective water management strategies are critical to achieving a balance between hydrogen production and ecological health.”
Challenges and Barriers in Hydrogen Production
Hydrogen production through water electrolysis holds great promise for the transition to sustainable energy sources. However, various challenges remain that need careful examination. Addressing these challenges is not just about technical prowess but involves economic dynamics, regulatory frameworks, and material science. By understanding these barriers, stakeholders can better navigate the complexities of hydrogen production, ensuring it contributes effectively to the energy landscape.
Economic Viability
Cost of Electrolysis Systems
The cost of electrolysis systems plays a pivotal role in determining the feasibility of hydrogen production. High capital expenditures associated with these systems can hinder wider adoption. These systems require significant investment in components like electrodes, electrolytes, and energy sources.
A notable characteristic of entry-level systems is their relatively low price compared to advanced models. However, they often compromise on efficiency. On the other hand, more sophisticated designs, while pricier, offer improved efficiency and durability, making them a better long-term investment.
The unique feature of these systems is the trade-off between initial costs and operational savings. For instance, systems utilizing advanced polymer membranes tend to be more efficient but demand higher upfront costs. Thus, stakeholders must weigh the benefits against the financial burden to determine the most suitable approach for their specific contexts.
Market Competitiveness with Other Methods
When comparing the market competitiveness of electrolysis with alternative methods of hydrogen production, several factors are at play. Traditional methods, such as steam methane reforming, benefit from established infrastructure and lower immediate costs. These methods often leave a larger carbon footprint, presenting a compelling counter-argument for electrolysis, particularly when powered by renewable energy sources.
The key characteristic of electrolysis is its potential to produce hydrogen with minimal emissions. This aspect positions it as a more environmentally friendly choice in the long run, even if it’s initially less competitive on price points. The unique feature of this competitiveness lies in the growing environmental regulations and carbon pricing initiatives, which may push industries towards cleaner production methods.
Yet, challenges remain in scaling up electrolysis to match the volumes produced by conventional techniques. The limitations in current infrastructure and market acceptance can stall progress unless addressed proactively.
Technological Limitations
Long-Term Durability of Materials
Long-term durability of materials used in electrolysis is a crucial factor that can hinder progress in hydrogen production. The electrodes and membranes are exposed to harsh conditions, which can lead to degradation over time.
A key characteristic of these materials is their susceptibility to corrosion and fouling, complicating maintenance routines and increasing operational costs. Innovations in materials, such as using new alloys or coatings, are being explored to enhance durability, but many solutions are still in their infancy.
Uniquely, this challenge impacts not only system maintenance but also safety and efficiency. A system that degrades too quickly will not only require more frequent replacements but could also lead to unintended production halts, limiting overall output potential.
Scaling Up Production Techniques
Scaling up production techniques in electrolysis presents a major hurdle. The complexity of replicating small-scale successes on a larger scale is daunting. Factors such as energy source availability, system logistics, and economic factors all intertwine to create a challenging landscape for scale-up.
The key characteristic in this context is the need for robust infrastructure and efficient supply chains to ensure that production can increase without significant resource waste. Developing modular systems that can be easily deployed in various settings may offer a path forward.
Moreover, a notable unique feature is the potential for decentralized production. It allows production closer to the point of use, reducing transport costs and energy losses. However, this concept's viability largely depends on technological advancements and economic incentives.
"Understanding these challenges is the first step in transforming hydrogen from a theoretical solution to a practical energy source."
In closing, addressing these challenges requires collaboration among industry players, researchers, and policymakers to unlock hydrogen's potential as a clean energy vector. Clear strategies focused on economic indicators and technological improvements are necessary for a sustainable hydrogen economy.
Future Prospects of Hydrogen Economy
Understanding the future prospects of hydrogen economy is not just an academic exercise; it carries far-reaching implications for how we envision energy production and sustainability in the coming decades. As nations strive to meet increasingly rigorous climate goals, the role of hydrogen as a clean energy carrier cannot be understated. Its ability to serve multiple sectors—transportation, industry, and even grid management—makes hydrogen a versatile player in the renewable energy landscape. Furthermore, innovations in technology and supportive policies will drive this transition, thereby enhancing economic viability and public acceptance of hydrogen solutions.
Innovations in Electrolysis Technology
Advancements in Electrode Materials
When we dig into advancements in electrode materials, the conversation often swirls around efficiency and durability. The key characteristic here is the development of catalysts that maximize hydrogen output while minimizing energy loss. For example, materials like platinum and iridium have been the gold standard due to their excellent catalytic properties. However, the race is on to find cheaper alternatives such as transition metal dichalcogenides that can withstand the corrosive environments typical in electrolysis setups. These innovations are essential for reducing the overall costs associated with hydrogen production and promoting wider adoption.
The unique feature of these new materials lies in their scalable production and comparable performance to traditional catalysts. While they may not yet match the performance metrics of precious metals, they offer significant advantages such as lower costs and reduced resource dependency. This aspect is crucial as we aim for a future where hydrogen production is not a luxury for wealthy nations but an accessible option worldwide.
Integration with Renewable Energy Systems
Another pivotal area is the integration with renewable energy systems. It represents a win-win situation where you can use surplus energy generated from solar or wind to produce hydrogen through electrolysis. This approach not only smooths out the intermittency issues associated with renewables but also provides an efficient way to store energy. The key characteristic here is flexibility—integrating hydrogen production with renewable sources can optimize energy usage based on real-time supply and demand.
The unique aspect is that it allows for the transformation of energy into a storable and transportable form. While the initial setup may require significant investment, the long-term environmental benefits and energy security may outweigh these costs. The advantages of this synergy are manifold, helping nations transition more rapidly towards sustainable energy goals and reduce dependency on fossil fuels.
Policy and Regulatory Frameworks
Global Hydrogen Strategies
As governments around the world recognize the potential of hydrogen, the establishment of coherent global hydrogen strategies emerges as a cornerstone for fostering this sector. The beauty of these strategies is they often align with broader decarbonization goals across multiple sectors. By creating frameworks that encourage research and investment, countries can support innovation while also establishing standardized practices that facilitate large-scale adoption.
The unique features of these strategies are they usually focus on international cooperation, data sharing, and commissioning large-scale pilot projects. They can also help level the playing field for emerging economies, allowing them to participate in the hydrogen revolution without being sidelined by technology gaps. However, the limitations of these frameworks often stem from regulatory inertia and varying levels of commitment across nations.
Subsidies and Incentives for Development
Lastly, discussing subsidies and incentives for development is crucial to catalyze the hydrogen economy. Government programs can lower the entry costs for hydrogen production, making the market more attractive for potential investors. Financial support can range from tax breaks to direct funding for research and development.
The primary feature of these incentives is their ability to accelerate innovation. When developers know they have a safety net, they are more likely to experiment with new technologies and business models. This, in turn, can lead to breakthroughs that might not be possible in a more conservative funding environment. Still, significant challenges remain, particularly with ensuring that these programs are equitable and that smaller players receive the support they need in order to innovate and compete.
"The transition to a hydrogen economy is not just about the technology; it's also about the policies that guide its development."