GFP Live Cell Imaging: Insights and Innovations
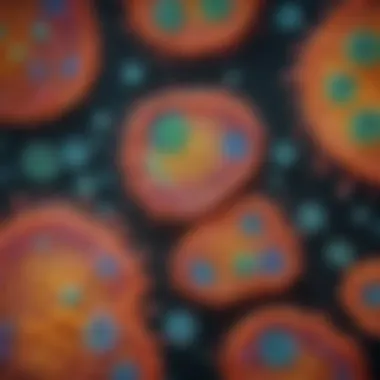
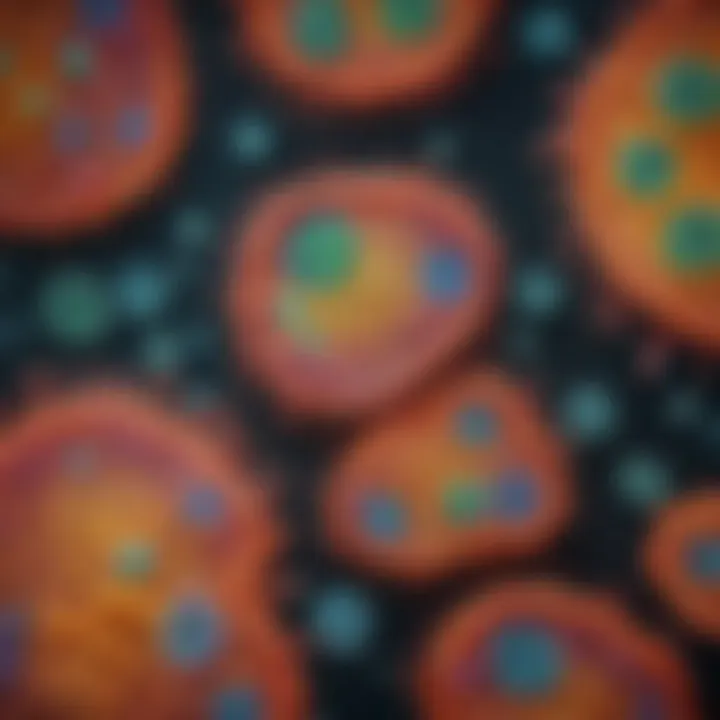
Overview of Research Topic
Brief Background and Context
Green fluorescent protein, or GFP, was initially discovered in the jellyfish Aequorea victoria. This protein provides a powerful tool for studying live cells due to its unique fluorescent properties. Over the years, GFP has evolved, with various mutants being developed to enhance brightness and spectral range, thus broadening its applications in cellular biology and related fields. Researchers recognized that by tagging proteins of interest with GFP, they could visualize and monitor dynamic biological processes in real-time.
Importance in Current Scientific Landscape
GFP live cell imaging represents a significant scientific advancement. It allows researchers to observe cellular behaviors, interactions, and changes as they occur. The value of this technique can be seen across various disciplines, including neuroscience, where scientists use it to track neuronal activities, and medical research, where it assists in understanding diseases at a molecular level. By facilitating these observations, GFP imaging transforms how scientists conduct experiments and interpret results, providing insights that were previously difficult or impossible to achieve.
Methodology
Research Design and Approach
The study and application of GFP live cell imaging require a systematic approach. Researchers start by designing experiments that clearly outline their goals and hypotheses. This includes selecting appropriate GFP variants that best suit their specific applications. The integration of GFP into the target cells is often achieved through genetic engineering techniques, such as plasmid transfection or viral transduction.
Data Collection Techniques
Data collection in GFP imaging primarily involves advanced microscopy techniques, such as fluorescence microscopy, confocal microscopy, and two-photon microscopy. These methods enable researchers to capture high-resolution images of living cells, with various imaging modalities tailored for different aspects of cellular dynamics. The images obtained can be analyzed through software that quantifies fluorescence intensity and tracks movement and localization. Continuous monitoring allows for the observation of real-time processes, such as cell division, migration, and interaction with other cells.
The ability to visualize cellular processes in real-time has revolutionized biological research, providing insights that enhance our understanding of life at the cellular level.
Prelude to GFP Live Cell Imaging
Green fluorescent protein (GFP) live cell imaging has emerged as a fundamental technology in biolological research, providing a unique window into the dynamic processes of living cells. The capacity to visualize cellular components and dynamics in real-time has revolutionized our understanding of cellular behavior, protein interactions, and signaling pathways. This section will explore the historical context of GFP development and its significance in the broader spectrum of imaging techniques.
Historical Background
The journey of GFP began with its discovery in the early 1960s in the jellyfish Aequorea victoria. Initially, it was a curiosity of nature, but by the late 1990s, scientists harnessed its properties for research. The pivotal work was done by Osamu Shimomura, who, in collaboration with Martin Chalfie and Roger Tsien, successfully developed GFP as a marker for gene expression. Their collective work led to a breakthrough for live cell imaging and earned them the Nobel Prize in Chemistry in 2008. The adaptability of GFP for tagging proteins within cells offered unprecedented insights into cellular function whereas traditional staining techniques fell short of this live analysis. Thus, GFP's inception marked a significant milestone in cell biology and research methodologies.
Defining GFP and Its Importance
GFP is an intrinsically fluorescent protein that emits green light when exposed to ultraviolet or blue light. Its unique properties, such as brightness and stability, make it a powerful tool in live cell imaging. The ability to label proteins, cells, and even entire organisms with GFP enables researchers to visualize complex processes in real-time.
The importance of GFP extends beyond its optical properties. It has greatly enhanced our capacity to study protein localization, interactions, and dynamics. Moreover, the versatility of GFP has led to the development of various fluorescence techniques, impacting fields like molecular biology, genetics, and pharmacology.
"GFP has transformed the landscape of cellular research, allowing for insights previously deemed impossible."
In summary, the introduction of GFP into biological research has not only enhanced imaging capabilities but has also fostered novel experimental designs, allowing researchers to probe the intricacies of life at a molecular level. The implications of GFP live cell imaging continue to resonate throughout the biological sciences as it reveals the hidden complexities of cellular mechanisms.
Mechanism of GFP Fluorescence
Understanding the mechanism of GFP fluorescence is crucial in the study of biological processes. The ability to visualize cellular functions in real-time has revolutionized research. It enables scientists to observe dynamic processes such as protein localization, cellular signaling, and overall cellular behavior. This section focuses on two main aspects of GFP fluorescence: its structural composition and the excitation and emission properties.
Structural Composition of GFP
Green fluorescent protein (GFP) originates from the bioluminescent jellyfish Aequorea victoria. Its unique structure allows it to emit a bright green light when exposed to ultraviolet or blue light. The protein consists of 238 amino acids forming an 11-stranded beta-barrel that encapsulates a chromophore, which is the light-emitting component. The chromophore is formed from a modified version of the amino acid tyrosine, and it undergoes a series of reactions once synthesized within the folds of the protein.
One significant property of GFP is its ability to withstand harsh cellular environments. The tight packing of the beta-barrel protects the chromophore from quenching, thus maintaining fluorescence intensity. This structural stability is vital for prolonged observations during live cell imaging.
Excitation and Emission Properties
The fluorescence of GFP arises from its characteristic excitation and emission properties. The excitation wavelength typically falls within the range of 395 to 485 nm, which means GFP absorbs photons in this spectrum. When excited, GFP molecules transition from a ground state to a higher energy state. Upon returning to a stable state, they emit light at around 507 nm, producing the green fluorescence that is seen during microscopy.
The efficiency of fluorescence is defined as the ratio of emitted photons to absorbed photons. High efficiency is essential for precise measurements in studies of protein dynamics.
Variations in GFP exist, including enhanced GFP, which offers brighter and more stable fluorescence, making it more suitable for prolonged imaging sessions. The choice of the specific GFP variant can significantly influence the success of experiments, depending on the biological context and imaging requirements.
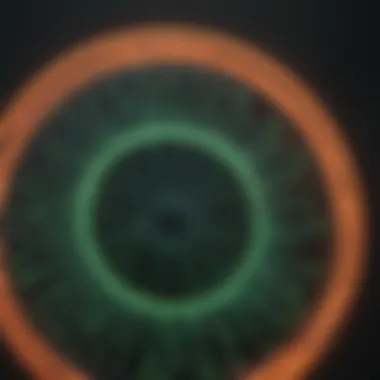
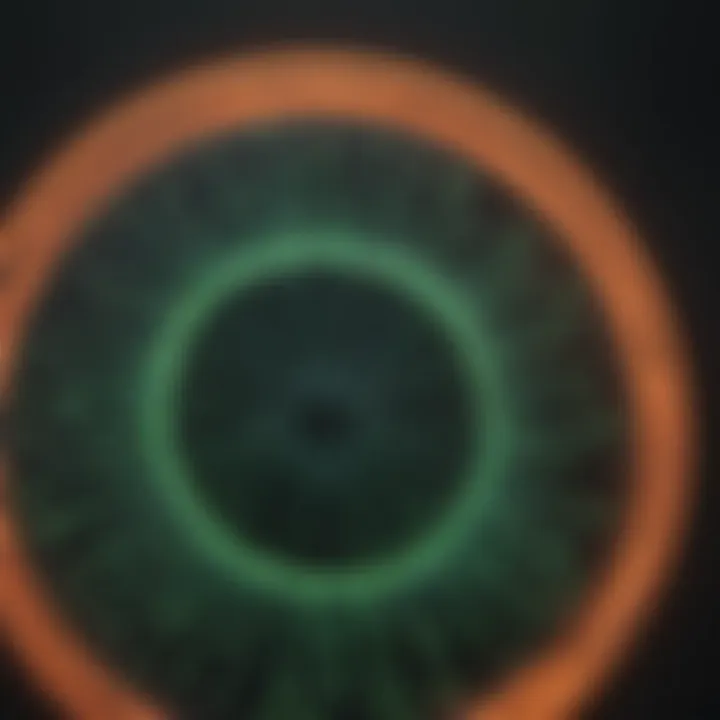
Overall, the mechanisms of GFP fluorescence underscore its utility in cell biology. They highlight the factors that contribute to its effectiveness in visualizing live cells, providing insights that are critical for understanding complex biological systems.
Techniques in Live Cell Imaging
The techniques used in live cell imaging are essential for understanding dynamic biological processes at the cellular level. They enable researchers to visualize and analyze cellular behavior in real-time. This section will elaborate on three core techniques: Fluorescence Microscopy, Confocal Imaging, and Total Internal Reflection Fluorescence Microscopy (TIRF). Each method has its benefits and drawbacks, suited for specific experimental needs. By employing these techniques effectively, scientists can obtain valuable insights into cellular mechanisms, which is crucial for various fields in biological research.
Fluorescence Microscopy
Fluorescence microscopy is a powerful tool widely used in live cell imaging. It takes advantage of fluorescent proteins, such as GFP, to visualize cellular components. The basic principle involves exciting fluorescent molecules with a specific wavelength of light, which in turn emits light at a longer wavelength. This allows researchers to observe structures that are typically invisible under standard light microscopy.
Key aspects of fluorescence microscopy include:
- High Sensitivity: This technique can detect low levels of fluorescence, making it possible to visualize small structures and proteins.
- Multiple Labels: Different fluorescent tags can be used simultaneously, facilitating the study of multiple proteins or cellular structures in a single sample.
- Dynamic Imaging: Fluorescence microscopy enables the observation of live cells over time, allowing for the analysis of dynamic processes like cell division and migration.
However, this approach also has limitations, including photobleaching and potential toxicity, which can impact the live cell's health.
Confocal Imaging
Confocal imaging enhances fluorescence microscopy by using a spatial pinhole that eliminates out-of-focus light. This results in images with higher resolution and contrast. It is especially valuable for studying three-dimensional structures of cells.
The advantages of confocal imaging are:
- Improved Resolution: By focusing on a single plane of the sample, confocal microscopy achieves much greater clarity.
- Optical Sectioning: This allows researchers to collect information from specific layers of the sample, leading to better three-dimensional reconstructions.
- Multichannel Imaging: Confocal systems can simultaneously capture different wavelengths, enabling complex multi-label studies.
Yet, confocal imaging can be more time-consuming and often requires more intensive illumination, which may lead to phototoxic effects on live specimens.
Total Internal Reflection Fluorescence Microscopy (TIRF)
TIRF microscopy is a unique technique that exploits the evanescent wave principle. By illuminating the sample at a critical angle, TIRF selectively excites fluorophores within a thin section close to the coverslip. This allows for exceptional detail of the membrane and cellular processes occurring at the interface between the cell and the substrate.
Some important features of TIRF include:
- Superficial Imaging: TIRF only illuminates a thin slice of the sample, minimizing background noise and improving the signal-to-noise ratio.
- Real-time Analysis: It facilitates the observation of rapid processes such as vesicle trafficking or receptor interactions in vivo.
- Reduced Phototoxicity: Since only a small volume of the sample is illuminated, TIRF can reduce the harmful effects on living cells compared to more intensive techniques.
Nonetheless, TIRF is limited to events occurring near the coverslip and may not provide comprehensive insights into the entire cell.
"The integration of these imaging techniques has revolutionized our ability to explore cellular dynamics, leading to new discoveries in biology and medicine."
In summary, the techniques in live cell imaging provide essential tools for understanding the complexities of cellular behavior. Each method presents unique advantages and practical considerations, which researchers must evaluate based on their specific objectives.
GFP Tagging Strategies
GFP tagging strategies are critical for harnessing the full potential of green fluorescent protein in live cell imaging. These strategies facilitate the monitoring of dynamic processes within cells, allowing researchers to elucidate the functions and interactions of proteins in real time. Effective tagging can also minimize the impact of the inserted tag on the natural behavior of proteins, which is crucial for obtaining accurate and meaningful data.
The key benefit of employing GFP tagging is the ability to visualize proteins without disrupting cellular activities. This capability enables a deeper understanding of cellular dynamics and mechanisms. Considerations in tagging include the choice between C-terminal and N-terminal tagging, the possibility of using dual-tagged constructs, and the selection of fluorescent protein variants that best suit specific applications.
GFP tagging enhances live cell imaging by allowing real-time observation of cellular processes.
C-terminal and N-terminal Tagging
C-terminal and N-terminal tagging represent two main approaches for attaching GFP to proteins of interest. C-terminal tagging involves fusing the GFP moiety to the end of the protein. This approach can be advantageous when the C-terminus is less critical for protein function. On the other hand, N-terminal tagging integrates GFP at the beginning of the protein sequence. This method is often preferred when the N-terminus is not vital for the protein's activity.
The choice between the two methods depends on the specific protein being studied. It is essential to consider the role of the tagged protein within the cellular context. Researchers often conduct preliminary tests to determine which tagging strategy results in functionality preservation while still allowing for effective visualization.
Dual-Tagged Constructs
Dual-tagged constructs involve fusing a second fluorescent protein to the target protein alongside the GFP tag. This approach expands the possibility of obtaining more complex data about protein interactions, localization, and dynamics. By using two different fluorescent tags, researchers can monitor multiple proteins or even different states of the same protein simultaneously.
Implementing dual tagging can provide insights into protein interactions that might not be evident when using a single tag. For instance, when studying protein-protein interactions, dual tagging allows visualization of both proteins in the same cell under varying conditions. This strategy can be especially important in understanding complex signaling pathways and cellular communications.
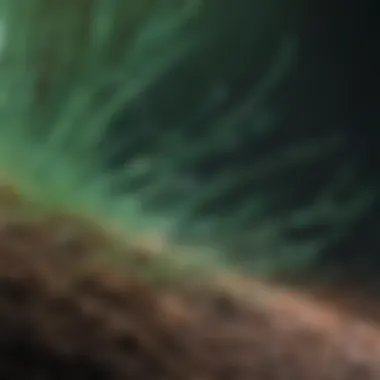
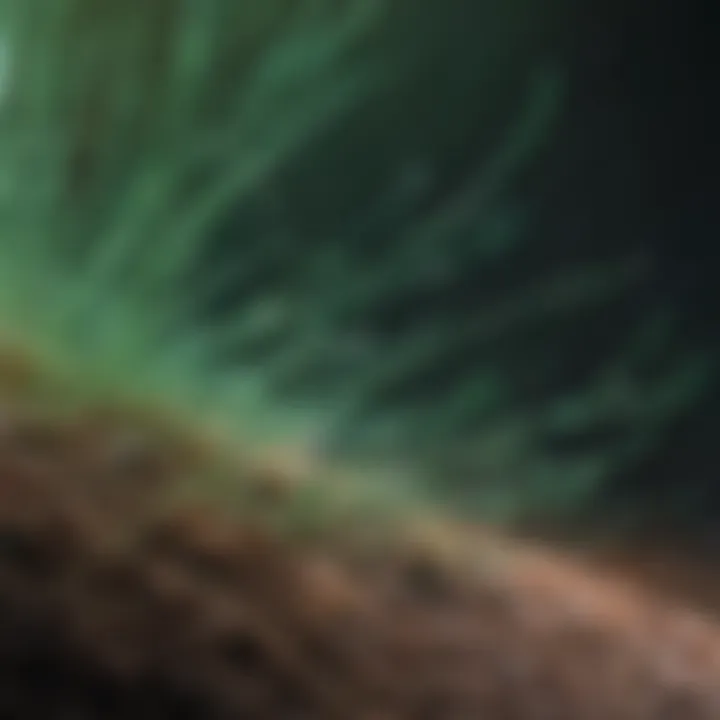
Fluorescent Protein Variants
Fluorescent protein variants expand the capabilities of live cell imaging by offering a diverse range of spectra, brightness, and photostability. Different variants of GFP, like EGFP (enhanced green fluorescent protein), mCherry, and mNeonGreen, provide options depending on the specific needs of the study. For example, if researchers require simultaneous imaging of proteins emitting different wavelengths, using multiple fluorescent variants can be beneficial.
Moreover, newer variants may exhibit improved properties such as increased brightness and reduced tendency for photobleaching, both critical for long-term imaging of live cells. Selecting the appropriate variant can depend on factors such as the cellular environment, the depth of tissue being imaged, and the experimental design.
In summary, GFP tagging strategies play a vital role in live cell imaging, allowing scientists to investigate the behavior and interactions of proteins within cellular contexts. Understanding the choices between C-terminal and N-terminal tagging, alongside the benefits of dual-tagged constructs and fluorescent protein variants, enables researchers to design effective imaging experiments.
Quantitative Analysis in Live Cell Imaging
Quantitative analysis plays a crucial role in live cell imaging, particularly when utilizing green fluorescent protein (GFP) technology. This aspect of GFP imaging allows researchers to obtain precise numerical data about various cellular processes. With the increasing complexity of biological systems, the need for accurate measurements has never been more important. Quantitative analysis allows for the comparison of experimental results, leading to more reproducible and reliable findings.
The benefits of quantitative analysis are manifold. It enables researchers to go beyond simple visual observations. Instead, they can capture dynamic biological processes and analyze the data statistically. This leads to insights that help define the mechanisms underlying cellular behavior. Moreover, quantitative methods can enhance the utility of GFP in diverse research contexts, such as drug discovery and genetic studies, where quantification is critical.
Considerations around quantitative analysis in live cell imaging include sensitivity and specificity in measurement techniques. Issues like photobleaching, which can alter fluorescence intensity, should be taken into account when designing experiments. Proper controls and calibrations are essential for obtaining valid data, which not only helps in determining concentrations of cellular components but also in tracking their dynamics over time.
Fluorescence Intensity Measurements
Fluorescence intensity measurements are fundamental in quantitative analysis. This technique involves capturing the brightness emitted by the GFP tags to represent protein localization and abundance. These measurements can provide insights into protein interactions, concentrations, and dynamics. Researchers can analyze intensity levels over time or under varying environmental conditions.
Key factors affecting fluorescence intensity include:
- Excitation power: Higher power can lead to increased brightness but may also accelerate photobleaching.
- Cellular conditions: For instance, the presence of proteins that might quench fluorescence can influence readings.
- Detection settings: Calibration of the imaging system is essential to reduce variability.
Overall, fluorescence intensity measurements equipped with the right controls can yield precise and meaningful data, crucial for understanding cellular processes.
Bioinformatics Approaches
Bioinformatics is increasingly vital in the context of GFP live cell imaging. With the vast amount of data generated in imaging experiments, computational tools are necessary to process and analyze this information effectively. Bioinformatic approaches enable the integration of imaging data with other biological data, providing a multifaceted view of cellular activities.
Researchers can use several bioinformatics methods, such as:
- Image analysis software: Tools like ImageJ or Fiji can quantify and analyze images to extract useful data.
- Statistical modeling: This helps in interpreting data trends and validating findings statistically.
- Machine learning algorithms: These can analyze large data sets to identify patterns not easily observed through standard methods.
Applications of GFP Live Cell Imaging
The applications of GFP live cell imaging stand at the forefront of modern biological research. These applications are diverse and extend across various fields, allowing scientists to investigate intricate cellular processes in real-time. This section delves into the significance of these applications, discussing specific elements, benefits, and key considerations.
Studying Protein Dynamics
GFP live cell imaging provides insightful data on protein dynamics. By tagging proteins with GFP, researchers can visualize their behavior within living cells. This capability enables the observation of protein interactions, movement, and localization over time. Understanding protein dynamics is crucial as it underpins many biological processes, including enzyme activity and cellular signaling. The ability to monitor these changes can elucidate mechanisms of disease and inform drug development strategies.
Cell Signaling Pathways
Another significant application of GFP live cell imaging is its role in studying cell signaling pathways. Live imaging allows scientists to observe how signals propagate within cells and the resulting activity at specific cellular locations. For instance, by tagging key proteins involved in signaling cascades, researchers can visualize how these proteins interact during signaling events. This extends our understanding of cellular responses to external stimuli and contributes to the comprehension of disorders such as cancer, where signaling pathways often become dysregulated.
Applications in Neuroscience
In neuroscience, the use of GFP tagging has been transformative. It facilitates the study of neuronal behavior, synaptic dynamics, and neural network activity in living organisms. For example, the observation of synaptic vesicles during neurotransmitter release can unveil critical insights into how communication occurs within the brain. Additionally, by tagging specific neuron types, researchers can trace their development and function in the context of the whole nervous system, providing a more comprehensive view of brain function and disorder.
Investigating Pathogen Behavior
GFP live cell imaging is also instrumental in investigating pathogen behavior. By labeling pathogens with GFP, scientists can track their interactions with host cells and understand their life cycles. This application is particularly relevant in studying infectious diseases, where understanding the dynamics of pathogen entry and replication can inform treatment strategies. It allows for real-time observation of immune responses as well, shedding light on how cells respond to infections.
In summary, the applications of GFP live cell imaging offer profound insights into the dynamic processes governing biological systems, presenting opportunities for advancements in research, medicine, and our broader understanding of life itself.
The integration of GFP live cell imaging techniques into various research fields demonstrates its versatility and importance. It not only enhances our comprehension of fundamental biological mechanisms but also supports the development of targeted therapies and innovative research methodologies.
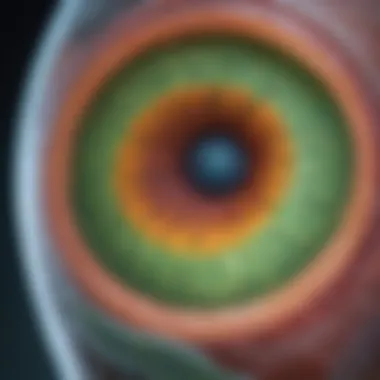
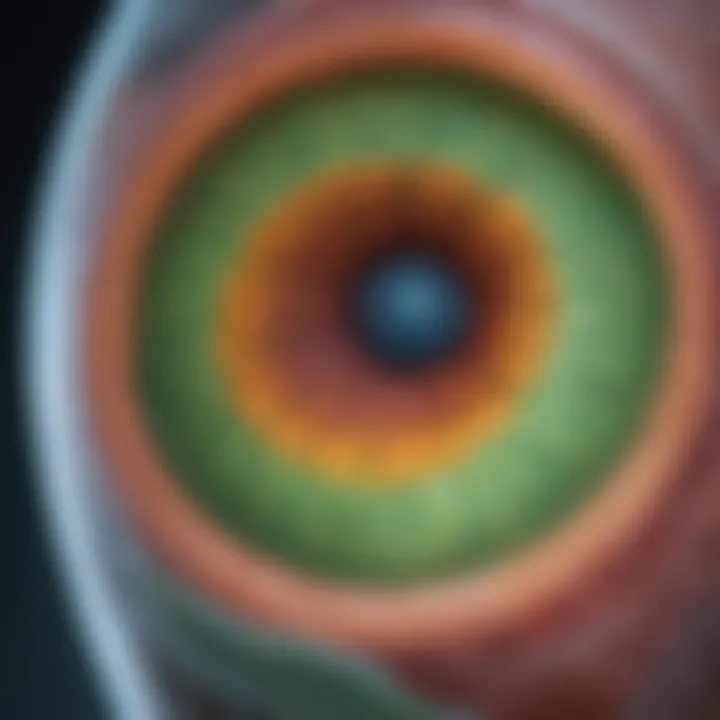
Challenges in Live Cell Imaging
The advancement of GFP live cell imaging presents significant opportunities for biological research. However, it is not without its challenges. Understanding these challenges is crucial, as they can limit the interpretative power of experimental results. Here, we will discuss critical concerns such as photobleaching, phototoxicity, and the cellular environment. Addressing these will inform future research and enable more accurate conclusions.
Photobleaching and Phototoxicity
Photobleaching occurs when a fluorescent molecule loses its ability to fluoresce following prolonged exposure to a strong light source. This is a common problem in live cell imaging and can impair the quality of data collected during experiments. As the fluorescent signal diminishes, it complicates the analysis of dynamic processes such as protein movement or interactions within cells.
Phototoxicity also poses a risk. High-intensity light required for imaging can lead to damage in live cells, potentially altering their behavior or viability. The effects can range from temporary functional impairment to irreversible cell death. These factors must be carefully managed to avoid compromising experimental outcomes.
To mitigate these issues, researchers may:
- Reduce light exposure by using intensity-modulated imaging techniques.
- Implement alternative fluorescent markers with higher photostability.
- Use appropriate imaging frequency to maintain cell health while collecting sufficient data.
"The balance between illumination intensity and observation time is essential for reliable GFP imaging results."
This nuanced approach allows researchers to continually adapt their imaging strategies as the light environment changes, preserving the integrity of live cell analyses.
Cellular Environment Influences
The cellular environment can significantly influence the behavior of fluorescent proteins and the accuracy of imaging techniques. Factors such as pH, temperature, and ionic concentrations can affect the stability and function of GFP. These variables contribute to the complexity of interpreting fluorescence signals.
Moreover, the cellular context itself—such as the presence of other biomolecules—can also influence fluorescence emissions. Interaction with other proteins or molecules can alter the folding and stability of the GFP tag, leading to variability in the signals captured during experiments.
Understanding the cellular environment entails:
- Conducting pre-experimental assessments of the cellular conditions.
- Using controls to measure baseline fluorescence in varying conditions.
- Considering the appropriateness of specific GFP tags in different cellular contexts.
This knowledge can help decipher complexities involved in fluorescence measurements and ensure that recorded data reflects true biological realities, rather than artifacts caused by external influences.
Future Directions in GFP Imaging
As the field of live cell imaging with green fluorescent protein (GFP) evolves, new opportunities for exploration and discovery continue to emerge. This topic is crucial not just for understanding existing applications but for paving the way for future research advancements. Enhanced techniques and novel engineering methods can significantly increase the resolution and effectiveness of GFP live cell imaging. Researchers are consistently looking for ways to refine imaging technologies and engineer improved fluorescent proteins, which may ultimately lead to groundbreaking findings in areas such as cell biology and medical research.
Advancements in Imaging Technologies
Recent advancements in imaging technologies are making a remarkable difference in GFP live cell imaging. Techniques such as super-resolution microscopy have improved the ability to visualize subcellular structures with high precision. Methods like STED (Stimulated Emission Depletion) and PALM (Photoactivated Localization Microscopy) can provide details at the nanometer scale. These developments allow researchers to study protein interactions and dynamics in real time under more physiological conditions.
Moreover, advancements in computational power have enabled improved image processing and analysis. Algorithms that maximize the use of data obtained during imaging can lead to enhanced image quality and faster processing times. This is particularly beneficial for experiments which require high-throughput imaging, like screening for drug efficacy or identifying cellular responses to stimuli.
Continued Innovations in Protein Engineering
Innovations in protein engineering are equally important for the future of GFP live cell imaging. The development of brighter and more photostable fluorescent proteins can address challenges such as photobleaching, which can hinder long-term imaging studies. New variants of GFP, such as mNeonGreen and mScarlet, offer a range of emission spectra which allows for multiplexed imaging. This enables the simultaneous observation of multiple targets, providing a more comprehensive understanding of biological processes.
Additionally, biosensors engineered with fluorescent proteins can provide real-time reporting of biochemical activities in live cells. This can include detecting changes in pH or ion concentrations that influence cell signaling. The trend towards designing GFP-containing constructs that react to specific cellular environments shows promise for elucidating dynamic cellular functions.
"The integration of advanced imaging and protein engineering opens new frontiers in our understanding of cellular mechanisms."
Epilogue
The conclusion of this article encapsulates the transformative role of GFP live cell imaging technology in biological research. This technique has fundamentally reshaped our understanding of cellular dynamics and molecular interactions within living organisms. One of the primary elements discussed is the advancement in imaging techniques that allow for real-time observation of proteins and cellular processes. As researchers navigate increasingly complex biological questions, these imaging tools provide clarity and granularity, which is necessary for accurate interpretations.
Also, the summary of key findings emphasizes that GFP imaging enhances the ability to study diverse biological phenomena. The ability to tag specific proteins enables scientists to visualize processes that were once merely theoretical. Researchers can explore intricate pathways in areas such as cell signaling and gene expression. Additionally, GFP imaging holds significant promise for applications in disease study, including cancer and neurodegenerative disorders.
Moreover, an important consideration addressed is the ongoing challenges faced in live cell imaging. Issues such as photobleaching and environmental effects can skew results, making it essential for future investigations to mitigate these factors. Collectively, understanding these challenges will produce more insightful and reliable outcomes in biological studies.
Summary of Key Findings
- GFP live cell imaging has revolutionized the visualization of protein dynamics within living cells, enriching our understanding of cellular functions.
- The technique provides crucial insights into molecular interactions, influencing areas like neuroscience and medical research.
- The ongoing technological advancements continue to refine imaging methods, ensuring high resolution and minimal interference with biological processes.
- Challenges such as phototoxicity are critical for researchers to address to improve experimental outcomes.
Implications for Future Research
The implications for future research in GFP live cell imaging are expansive and multifaceted. First, there is a clear need for advancements in imaging technologies. Innovations that enhance resolution and reduce photodamage can significantly increase the viability of long-term studies in live cells. Researchers are also encouraged to explore additional fluorescent protein variants. This exploration could lead to better tagging strategies, facilitating complex multi-category studies.
Moreover, bioinformatics plays a vital role in future research. Integrating computational tools with live cell imaging data will provide deeper insights into cellular behavior. A more refined analysis will enable researchers to formulate comprehensive models that can predict cellular responses under various conditions.