Exploring Photon Measurement in Quantum Mechanics
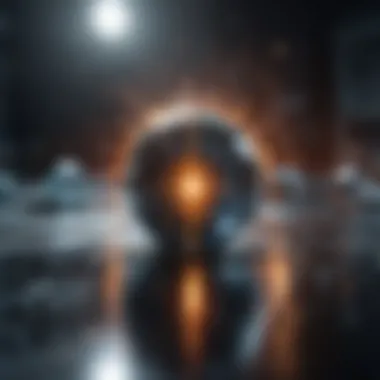
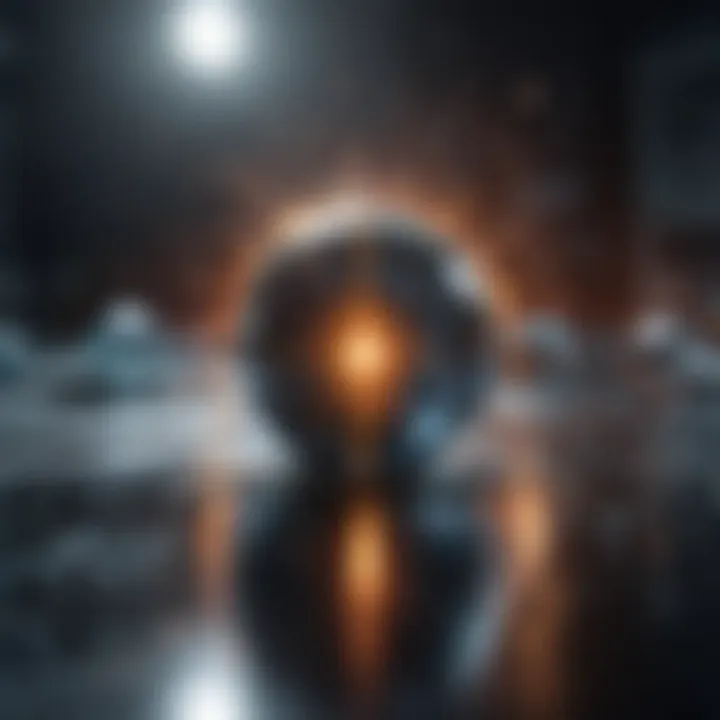
Intro
In the intricate realm of quantum mechanics, the study of photons stands as a cornerstone, intertwining physics, technology, and even philosophy. Photons, described as the fundamental particles of light, carry immense significance as they bridge our understanding of electromagnetic phenomena and the behavior of particles at microscopic scales. From the moment light hits our eyes to its instrumental role in advanced technologies like quantum computing, photons interact with our universe in ways both mysterious and enlightening.
This exploration into photon measurement delves into the processes that allow scientists to quantify, observe, and manipulate these elusive particles. With roots in the early 20th century, where Max Planck and Albert Einstein floated revolutionary ideas, the journey of photon measurement is marked by milestones reflecting profound changes in our comprehension of both light and matter. The implications extend far beyond theoretical musings—fields like telecommunications, photography, and even medical imaging rely heavily on advanced photonic measurement techniques.
As we ponder the relevance of photon measurement today, it’s clear that understanding how light behaves on quantum levels opens up new avenues in science and industry alike.
Overview of Research Topic
Brief Background and Context
The history of photon measurement is hardly linear. In the early days, physicists struggled to comprehend the dual nature of light—its capacity to act as both a wave and a particle. This dilemma sparked debates that would not find resolution until quantum theory offered a framework. The introduction of methods such as photoelectric effect measurements by Einstein laid the groundwork for the revelation that light can indeed behave as discrete packets, or photons.
As our technological capabilities evolved, so too did our measurement techniques. Early experiments relied on rudimentary equipment and direct observations. Modern techniques, however, harness complex apparatuses, enabling precision measurements with unprecedented accuracy.
Importance in Current Scientific Landscape
Photons are no longer merely subjects of theoretical inquiry; they are integral to numerous modern applications.
- Telecommunications: Optical fibers leverage light for high-speed data transmission, necessitating sophisticated measurement techniques to gauge signal integrity and fidelity.
- Medical Imaging: Technologies such as PET scans rely on photon detection to visualize metabolic processes within the body, bridging the gap between physics and medicine.
- Quantum Computing: The race towards practical quantum systems underlines the need for precise control and measurement of quantum states associated with photons.
In this context, photon measurement not only feeds into theoretical development but also fuels innovation across fields, making it a topic of substantial relevance.
Methodology
Research Design and Approach
This article's design is grounded in a comprehensive literature review that encapsulates both historical and contemporary perspectives. By synthesizing scholarly articles, white papers, and case studies, we aim to chart a course from early photon studies to cutting-edge applications. The methodology thus unravels the complexities surrounding photon measurement, seeking clarity in an often convoluted domain.
Data Collection Techniques
To gather relevant data and insights, this exploration embraces a multi-faceted approach:
- Literary Research: Engaging with academic journals and textbooks ensures a well-rounded understanding of photon theories and measurement techniques.
- Case Studies: Examination of specific applications in technology illustrates real-world implications of photon measurement methodologies.
- Expert Interviews: Conversations with physicists and engineers provide insider perspectives, enriching the narrative with practical experiences and insights.
In wrapping up this introduction, it becomes evident that as the world spins ever faster into the quantum age, understanding the mechanisms underlying photon measurement is crucial. We not only learn about the nature of light but also about the underlying principles of our reality.
Foreword to Photon Measurement
Photon measurement stands as a cornerstone in the realms of quantum mechanics and optics, illuminating the enigmatic behaviors of photons—those tiny, tantalizing particles of light that permeate our universe. Understanding how photons are measured is not just an academic exercise; it taps directly into the fabric of modern technology and scientific progress. As we plunge into this exploration, we must appreciate the broader implications of photon measurement.
In an age where advancements in areas like quantum computing and medical imaging rely heavily on precise photon measurement, grasping the intricacies of this topic opens new doors for students, researchers, and professionals alike. The multifaceted nature of photons, oscillating between being particles and waves, adds layers of complexity to their measurement, often requiring sophisticated techniques and equipment.
By delving into the history, definitions, and methods related to photon measurement, this article aims to equip readers with not only theoretical insights but also practical knowledge applicable to various fields. The topics ahead will cover the nature of light, the tools used for measurement, challenges faced, and the exciting applications stemming from our understanding of photons.
"Physics is like sex: sure, it may give some practical results, but that's not why we do it." – Richard P. Feynman
As we proceed, keep in mind the delicate balance between abstraction and practical implementation. The discussion will wind through the definitions of photons and their historical contexts before revealing how efforts to measure photons have evolved.
Definition of Photons
Photons are the elemental quanta of light and electromagnetic radiation. They are unique, massless particles that travel at the speed of light in a vacuum—roughly 299,792 kilometers per second. Each photon carries a specific amount of energy, which can be described by the equation:
[ E = h u ]
where ( E ) represents energy, ( h ) is Planck's constant, and ( \nu ) (nu) is the frequency of the electromagnetic wave. This relationship underscores how the energy of a photon is directly related to its frequency, giving birth to various phenomena observed in optics, such as the photoelectric effect and spectral distributions.
The quantized nature of photons translates into observable consequences, ranging from their role in supporting daily technologies, like lasers and LED lights, to more advanced concepts like quantum entanglement. Being both a particle and a wave, photons behave differently in various contexts, necessitating distinct measurement approaches based on the aspects being examined.
Historical Context
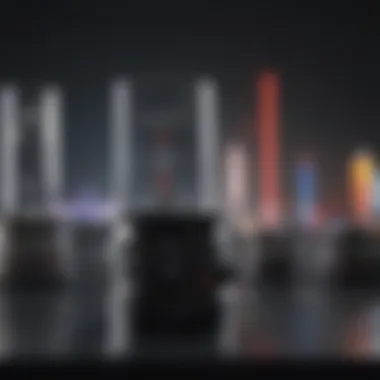
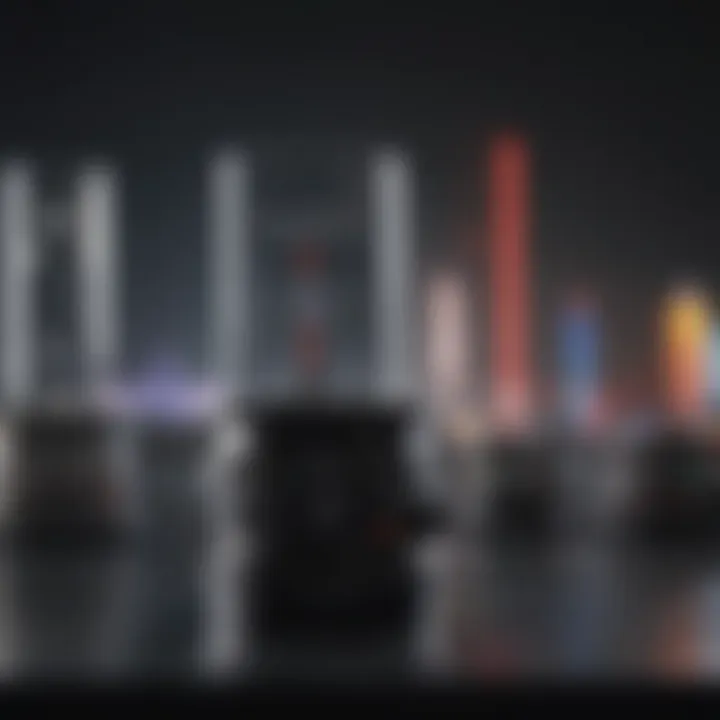
The journey to comprehend photons starts way back with the early scientists who sought to understand light. Before the concept of photons even entered the picture, theories revolved around light as a wave, as championed by Thomas Young in the early 1800s. His double-slit experiment provided compelling evidence of light's wave properties.
However, it wasn't until Albert Einstein's contributions in 1905 that light’s particle nature gained recognition. His work on the photoelectric effect demonstrated that light could indeed be quantized into discrete packets of energy—what we now call photons. This revelation marked a turning point, leading to the establishment of quantum mechanics as a major field of study.
Further developments unfolded throughout the 20th century, with figures like Niels Bohr and Max Planck expanding our understanding of light and its interactions. These foundational theories paved the way for modern technologies utilizing photon measurement, serving as a springboard for innovations in quantum computing and beyond. The historical context sheds light on how our grasp of photons matured, setting the stage for the intricate tools and methods we employ today in photon measurement.
The Nature of Light and Photons
Understanding the nature of light and photons is pivotal in our investigation of photon measurement. Light, in its essence, is a fundamental aspect of our universe, intertwining both art and science. Defining light merely as a visible spectrum would be too simplisitc. It comprises a vast array of wavelengths, each carrying distinct properties. In essence, the behavior of light is crucial for various applications, from photography to quantum computing and beyond.
Photons, the elementary particles of light, maintain a unique role in both physics and practical fields. They offer remarkable insights into quantum mechanics, which has reshaped our understanding of reality. When exploring photon measurement, it's essential to grasp how these tiny packets of energy interact with matter.
Wave-Particle Duality
One of the most captivating characteristics of light is its wave-particle duality. This duality challenges traditional perspectives on physics, where a clear-cut distinction between waves and particles might be anticipated. Instead, light displays behaviors of both. For example, it can exhibit properties like interference and diffraction, hallmarks of waves, while also releasing discrete packets, or photons, especially when energy is absorbed or emitted.
This duality is not just academic jargon; it has tangible implications for technology and science. Consider the use of lasers, which depend on coherent light waves to function. In contrast, devices like photodetectors rely on capturing individual photons. This dynamic flexibility allows diverse technologies to thrive, making wave-particle duality a cornerstone of light studies.
Moreover, the concept escalates our intuition regarding reality's underlying structure. If light can behave as both a wave and a particle, what does that mean about the nature of other entities in the quantum realm? Such contemplations provide fertile ground for advanced theoretical exploration. A famous thought experiment, the double-slit experiment, aptly illustrates this mysterious nature. It shows how light behaves differently when observed, grounding concepts that propel quantum physics forward.
Quantization of Light
Diving further into the nature of light, we encounter the quantization of light, which is foundational in appreciating photon measurement. In contrast to classical physics, which treats energy as a continuous entity, quantum mechanics proposes that energy occurs in quantized packets, or quanta.
Photons operate under this framework, allowing us to measure light's energy more accurately. The energy of a single photon is directly proportional to its frequency, expressed in the equation:
[ E = h imes f ]
where ( E ) denotes the energy, ( h ) stands for Planck’s constant, and ( f ) is the frequency of the photon.
This realization is particularly relevant in fields such as spectroscopy and telecommunications. For instance, precise calculations involving photons lead to advancements in fiber-optic communication systems, providing high-speed internet connections globally. In medical imaging, understanding the photon energy helps craft better diagnostic tools.
To summarize, grasping the nature of light and photons isn't merely an academic exercise. It's a foundation upon which both theoretical and practical advancements stand, allowing us to bridge the gap between the tangible world and the abstract concepts of quantum mechanics.
"The true nature of light transcends the boundaries of our imagination, leading us into realms where the classical meets the quantum, and where our understanding of the universe continues to deepen."
By becoming familiar with wave-particle duality and quantization, we can better appreciate the complexities and tools involved in measuring photons accurately. Recognizing these principles prepares us for a more profound inquiry into photon measurement techniques.
Measuring Photons: Techniques and Tools
In the intricate dance of quantum mechanics, measuring photons is akin to carefully counting beads on a delicate string. The techniques and tools utilized for photon measurement play an unmissable role in optical sciences and technology. As we delve deeper into this topic, we uncover how these methods not only enhance our understanding of light but also open the door to numerous practical applications.
Photo Detectors
Photo detectors serve as the first line of defense in the realm of photon measurement. These devices are designed to convert light into an electrical signal, thus facilitating the quantification and analysis of photons. Their importance cannot be overstated, as they are central to various fields such as telecommunications, medical diagnostics, and environmental monitoring.
Different types of photo detectors have been developed to meet specific needs. For instance, photomultiplier tubes (PMTs) are renowned for their high sensitivity to low light levels, making them ideal for applications in radiology and astrophysics. On the other hand, silicon photodiodes are widely used in consumer electronics due to their compact size and efficiency.
Advantages of photo detectors include:
- High response speed: Critical for applications like fiber optic communications, where rapid signal processing is essential.
- Versatility: Can be used across different wavelengths, allowing for a broad range of applications.
- Reliability: Many modern detectors feature enhanced noise reduction capabilities, improving measurement accuracy.
"Measuring light accurately requires tools that not only detect photons but also interpret them in meaningful ways."
Interferometry
Interferometry stands out as a sophisticated method for measuring photons, leveraging the wave nature of light. This technique involves superimposing light waves to create an interference pattern, which provides insights into very minute changes in distance or phase.
Traditional interferometry, such as Mach-Zehnder interferometry, utilizes beamsplitters to divide and later recombine light beams, effectively allowing scientists to observe changes in optical path length. Such precision makes it particularly valuable in fields like gravitational wave detection, where even tiny variations can signal monumental cosmic events.
Notable advantages of interferometry include:
- Precision measurement: Capable of detecting changes at nanometer scales, which is critical for advanced scientific research.
- Non-destructive testing: The nature of the technique allows measurements without altering the sample.
- Application breadth: From telecommunications to materials science, the applications are diverse and significantly impactful.
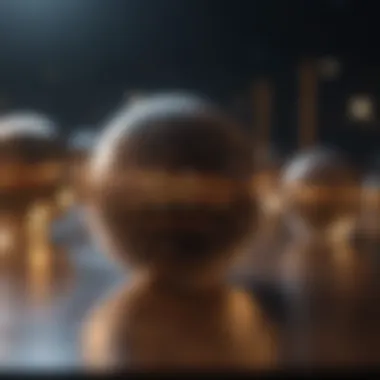
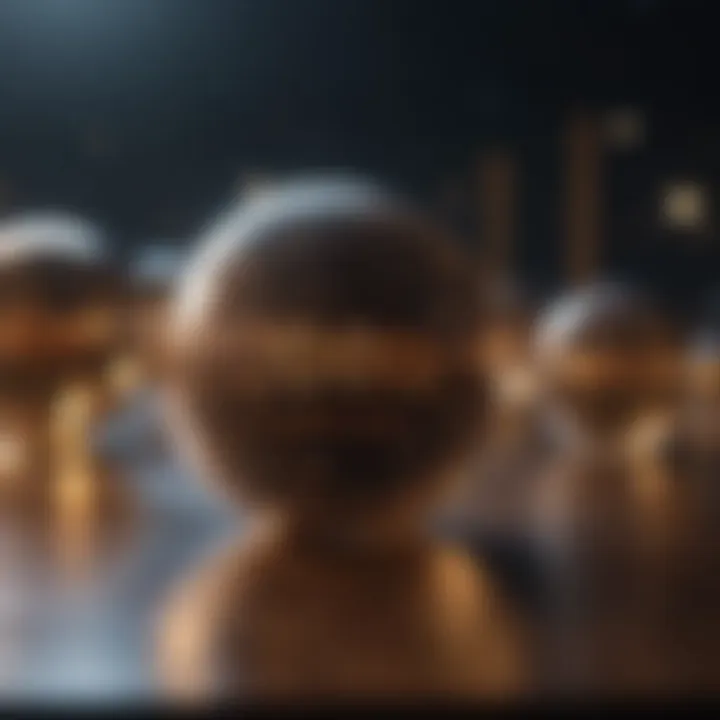
Single-Photon Sources
Single-photon sources take center stage when discussing photon measurement techniques. These sources are pivotal in quantum communications and quantum computing. The ability to generate and measure individual photons allows researchers to explore quantum phenomena without the noise typically associated with bulk photon measurements.
Devices like quantum dots and nitrogen-vacancy centers in diamond are leading technologies in generating single photons. Their importance lies not just in fundamental research but also in practical applications that could revolutionize secure communication methods.
Several key points highlight single-photon sources:
- Quantum entanglement: They facilitate experiments that test and utilize entangled states, essential for quantum cryptography.
- Low-light conditions: They help overcome challenges faced in low-light environments, enhancing measurement techniques.
- Preparation for future technologies: As quantum technology continues to advance, single-photon sources will be essential in building robust quantum networks.
The tools and techniques we have explored here underline the sophistication required in photon measurement. From the simple yet powerful principles of photo detectors, to the advanced methodologies of interferometry and the cutting-edge technology of single-photon sources, each plays a critical role in pushing the boundaries of light measurement. Without a doubt, understanding these techniques lays a firm groundwork for appreciating the complexities and possibilities within the world of quantum mechanics.
The Role of Quantum Mechanics in Photon Measurement
Understanding the intricacies of photon measurement is deeply tied to the principles of quantum mechanics. This branch of physics provides the framework through which we can discern not just the behavior of light, but also the phenomena that emerge when light interacts with matter. By exploring quantum concepts, particularly those related to measurement, we can unravel the complexities surrounding photons.
Measurement Problem in Quantum Mechanics
The measurement problem stands as one of the cornerstone issues in quantum mechanics. At its core, it grapples with how a quantum system transitions from a state of superposition—where multiple outcomes could occur—to a definite state upon observation.
In terms of photon measurement, this quandary is particularly poignant. Take, for instance, the famous double-slit experiment. When photons are not being observed, they can display wave-like characteristics, interfering with each other as if they occupy all possible paths simultaneously. However, once a measurement is taken, such as positioning a detector in front of the slits, the photons behave as particles, choosing one path while eliminating the wave nature entirely.
This phenomenon raises critical questions:
- What dictates the transition from potentiality to actuality in measurement?
- Is it the act of observation itself that influences the outcome?
Such inquiries delve into the philosophical implications of quantum mechanics, compelling scientists to reconsider their understanding of reality and existence. Theories like the Copenhagen interpretation suggest that reality becomes determined only through measurement, while alternatives like the Many-Worlds interpretation posit that all possible outcomes coexist in separate branches of the universe. Understanding these theories enriches our comprehension of how photons behave when measured and the underlying principles governing their nature.
Wave Function Collapse
Wave function collapse is an essential aspect of the measurement problem and is critical for interpreting the dynamics during photon measurement. The wave function, in its essence, is a mathematical representation that encapsulates all the potential states a particle could occupy. Its evolution is described by the Schrödinger equation until a measurement is made.
When photons are measured, such as detecting their position or momentum, the wave function collapses from a multitude of potential states to a singular specific outcome.
This concept raises further inquiries:
- What mechanisms are responsible for the collapse?
- Does collapse happen instantaneously, or is it a gradual process?
These questions are not just theoretical discussions but have practical repercussions in the field of quantum optics. It affects how we understand and utilize single-photon sources, which are crucial for advancements in quantum computing and cryptography. The very act of measurement changes the behavior of photons in a way that is not merely an idle observation; it's an interaction that redefines the properties of light itself.
"In quantum mechanics, every act of measurement alters the state of a system; thus, observation is an integral part of physical reality."
Exploring these themes further equips students, researchers, and professionals with a nuanced perspective on the fundamental nature of reality, ultimately illuminating the path for future innovations in photon-related applications.
Challenges in Photon Measurement
The measurement of photons presents a myriad of challenges, which are paramount in understanding not just the mechanics of light, but also its practical applications across various scientific fields. The importance of addressing these challenges cannot be overstated. They shape the capabilities of photon-based technologies, influencing future innovations, enhancing efficiency, and minimizing errors.
Detective Efficiency and Noise
Detective efficiency is a critical factor in the realm of photon measurement. It refers to the ability of measurement systems to accurately detect and quantify photons as they arrive. In practical terms, this denotes the ratio of detected photons to the total number of photons incident on the detection surface. In many scenarios, the environment introduces noise, which can considerably affect the accuracy of measurements.
Various types of noise can contribute to these challenges:
- Dark Noise: This type of noise emerges from the electronic components of a detector, even in the absence of light. The random fluctuations generated can mimic the signal of incoming photons, muddying the measurements.
- Photon Shot Noise: Intrinsic to the quantum nature of light, this noise presents a challenge due to the statistical variation in the arrival time and number of detected photons. As per the principles of quantum theory, even under optimal conditions, the detection is subject to unavoidable statistical fluctuations.
- Environmental Noise: External factors like temperature fluctuations and electromagnetic interference can further complicate the detection process.
The relationship between detective efficiency and noise is crucial for advancing optical technologies like quantum computing and medical imaging. Technological innovations aim to reduce noise levels while maximizing efficiency, which is a delicate balancing act.
Limitations of Current Techniques
Despite significant advancements in photonic technologies, current measurement techniques come with their own set of limitations. One notable aspect is the challenge of temporal resolution. Many detectors operate within a certain bandwidth and offers limited response to rapid changes in light intensity, which poses issues in applications demanding high-speed measurements.
Another limitation can be found in the quantification process. While some techniques can detect single photons, they struggle with ensuring accurate measurement when it comes to high-intensity light sources. This variability can lead to discrepancies in data, especially when high fidelity is required.
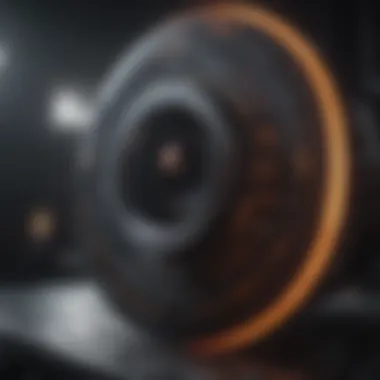
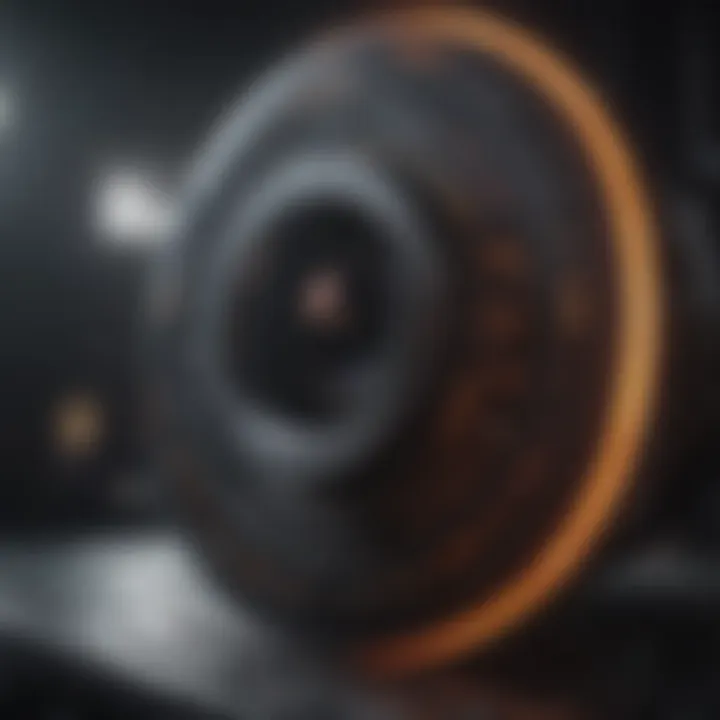
Moreover, the scaling of devices presents practical hurdles. Techniques that successfully measure photons under controlled laboratory conditions may not translate well to real-world scenarios where variables abound.
Applications of Photon Measurement
The realm of photon measurement holds significant relevance in a variety of scientific and technological fields. As we delve into the specific applications discussed in this article, it becomes clear that the understanding and manipulation of photons can lead to groundbreaking advancements. This is especially true when we consider how photon measurement plays a crucial role in three primary sectors: quantum computing, cryptography, and medical imaging. By examining these areas, we can appreciate the transformative potential and ongoing significance of photon measurement in today’s world.
Quantum Computing
Quantum computing is still a burgeoning field, and photons are at the heart of its operation. Utilizing the properties of photons, researchers can develop qubits that can exist in multiple states simultaneously, a phenomenon pivotal for quantum computing's power. This ability not only enhances computational speeds but also allows for complex problem-solving that classical computers struggle with.
Imagine a scenario where only one qubit is needed instead of dozens; this is made possible through the manipulation of individual photons with precision. As a result, computational tasks like optimization and simulation can be executed at unprecedented speeds.
Furthermore, photon-based quantum processors promise to reduce errors significantly compared to their traditional counterparts due to the quantum properties inherent in photons. Hence, the pursuit of efficient and reliable quantum computing solutions hinges on innovations in photon measurement.
Cryptography
The realm of cryptography has been revolutionized by the principles of quantum mechanics, particularly through the concept of quantum key distribution (QKD). In this system, the security of information is maintained through the laws of physics rather than traditional mathematical algorithms. When photons are used for transmitting keys, any attempt at eavesdropping can be detected, as measuring a quantum state alters it.
For instance, BB84 Protocol, one of the first QKD protocols, employs photon polarization states to encode bit values. If an unauthorized party tries to intercept the key during transmission, they inevitably disturb the polarization, thereby alerting the communicating parties about a potential breach. This layered security system leverages photon measurement to provide robust protection against cyber threats, imperative in an age where data breaches are rampant.
Medical Imaging
In the medical field, photon measurement has led to substantial advancements, particularly in imaging techniques. Technologies like Positron Emission Tomography (PET) scan and Optical Coherence Tomography (OCT) heavily rely on the measurement of photons for accurate diagnostics.
Photon measurement allows for high-resolution imaging that can reveal not only structural information but also functional metabolic processes within the body. For instance, PET scans utilize annihilation photons emitted when positrons collide with electrons in the body. The precise measurement of these photons enables doctors to observe the metabolic activity of tissues, aiding in early detection of diseases such as cancer.
Additionally, OCT utilizes coherent light to produce cross-sectional images of biological tissues. This technique measures the echo time delay of photons penetrating the tissue, providing detailed images with minimal invasive procedures. Thus, advancements in photon measurement continue to significantly contribute to enhanced medical imaging techniques, shaping how healthcare providers diagnose and treat various medical conditions.
Understanding how photons can be measured and applied is central to unlocking the marvels of quantum technologies and advancing modern medicine.
Overall, the applications of photon measurement are vast and hold incredible implications for both theoretical exploration and practical utility. Whether through reshaping quantum computing, securing communications, or revolutionizing medical imaging, the potential for photon measurement is both exciting and expansive.
Future Directions in Photon Measurement Research
The future of photon measurement holds a remarkable potential, not only for the field of quantum mechanics but also for various practical applications across science and technology. As researchers delve deeper into this area, they uncover layers of complexity and opportunity, leading to advancements that could revolutionize our understanding of light and its applications. There are specific elements worth highlighting, such as emerging technologies that promise to enhance measurement precision, and interdisciplinary approaches that could bridge gaps between different fields of study.
Emerging Technologies
New technologies are reshaping the landscape of photon measurement. For instance, the advent of quantum sensors has opened up avenues for unprecedented levels of sensitivity. These sensors utilize the quantum properties of photons to detect weak signals that traditional methods might miss. Some of the current trends include:
- Single-Photon Detectors: Innovations like superconducting nanowires are pushing the envelope in terms of sensitivity and speed, allowing for the detection of individual photons with minimal noise.
- Photonic Integrated Circuits (PICs): Integrating various photonic components on a single chip enhances performance and reduces costs. This technology is crucial for applications in telecommunications, where fast and reliable photon measurement is essential.
- Quantum Imaging Techniques: These methods not only measure light but also provide additional information about the spatial features of the light field, which could advance areas such as medical imaging, surveillance, and even astronomy.
This focus on technology where quantum mechanics and practical engineering meet will lead to breakthroughs that could change our understanding and utilization of photons.
Interdisciplinary Approaches
The challenges and possibilities within photon measurement do not exist in isolation. They often require insights from various fields, effectively blending theoretical physics with engineering, computer science, and even biology. An interdisciplinary approach can foster innovative solutions to some of the most pressing issues in photon measurement.
- Physics and Engineering: Collaborations between physicists and engineers can lead to the development of new instruments and techniques that push the boundaries of photon measurement.
- Data Science: The application of machine learning algorithms could help in processing and analyzing the vast amount of data generated by photon measurement experiments. This synergy can optimize detection systems by predicting photon behavior in complex environments.
- Life Sciences: By integrating photon measurement techniques into biophysics and biomedical engineering, researchers can create more precise imaging systems that offer insights into biological processes at the molecular level.
This confluence of disciplines epitomizes the need for a broader perspective in tackling photon measurement challenges.
"The future of photon measurement is an intricate tapestry woven from various scientific disciplines, each contributing unique threads of knowledge."
The Ends
In summing up this extensive examination, the concept of photon measurement serves as a cornerstone in both quantum mechanics and optics. The intricate interplay between theories and methodologies emphasizes the unique role of photons in understanding our universe. By dissecting the behaviors of light at such a granular level, researchers and professionals gain insights that transcend basic physical principles.
One key takeaway is how photon measurement acts as a gateway to advancements in technology and science. Here are a few notable points:
- Foundational to Quantum Mechanics: Photon measurements bring clarity to the complex behaviors associated with quantum states and wave functions. This clarity enhances our grasp of quantum mechanics, ultimately pushing us towards more profound discoveries.
- Practical Applications: They play a vital role across various domains such as quantum computing, cryptography, and medical imaging. The practical implications of mastering these techniques can lead to significant innovations in these fields.
- Ongoing Challenges: Despite the strides made, photon measurement does not come without its hurdles. Issues such as detector efficiency and noise continue to be points of contention, driving researchers toward finding solutions that can refine measurement techniques.
In a fast-evolving field like this, staying abreast of emerging technologies and interdisciplinary approaches is paramount. The future holds potential breakthroughs that could redefine our understanding of light and its interaction with matter.
Moreover, the dialogue between theory and practice reinforces the notion that photon measurement is not just about numbers or data. It delves deeper into how we conceptualize light itself, prompting us to rethink established models and embrace innovative approaches.
As we look ahead, one cannot overstress the importance of nurturing collaborations across scientific disciplines. Such partnerships can pave the way for new ideas and solutions, ensuring that the flow of knowledge continues unabated.
"To grasp the nature of light is to touch the fabric of reality itself."