The Intricacies of DNA Replication Explained
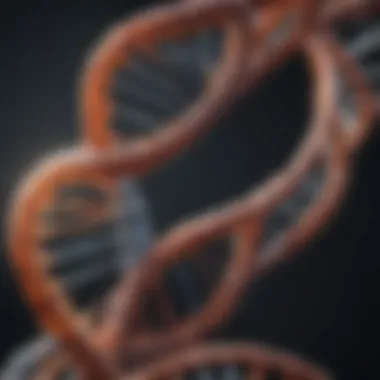
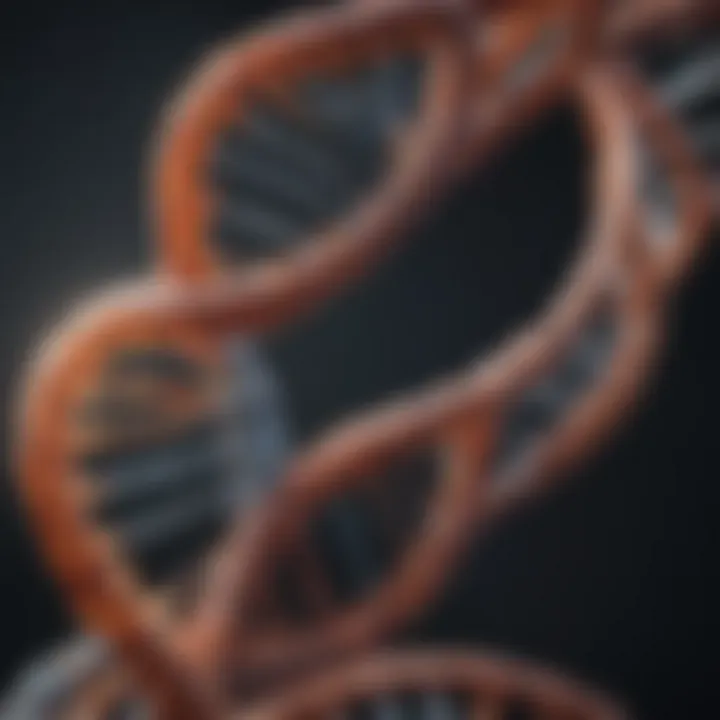
Intro
DNA replication is a crucial process in molecular biology, underpinning the continuity of life by allowing organisms to duplicate their genetic material. Understanding this process is essential for students, researchers, and professionals in various fields, including genetics, biochemistry, and biomedical sciences. The precision of DNA replication directly influences genetic stability, which is vital for the normal functioning of cells, preservation of traits across generations, and the potential for evolution.
In the coming sections, we shall delve into the complex journey of DNA replication, broken down into several stages. Each stage is marked by the involvement of specific enzymes and proteins that ensure each step is executed correctly. From the unwinding of the DNA helix to the synthesis of new strands, we will explore this fascinating biological phenomenon comprehensively.
In addition, we will address the importance of this process in the broader scientific landscape, including its ramifications for genetic diversity and the understanding of hereditary diseases. Through this exploration, it will become evident how various perturbations in DNA replication can lead to significant biological consequences, thereby influencing not just individual organismal biology but also population genetics as a whole.
Preface to DNA Replication
DNA replication is a crucial process in the life of all living organisms. It allows cells to make exact copies of their genetic material, ensuring that when cells divide, they pass on the correct and complete set of instructions to their offspring. Understanding this process is not just a fundamental topic in biology; it has far-reaching implications in fields such as genetics, biotechnology, and medicine.
The significance of DNA replication can be found in its role in genetic stability. A precise replication process ensures that genetic information remains unchanged as it gets transmitted through generations. Any errors in this mechanism could lead to mutations, which may cause diseases or affect the organism's ability to adapt.
Additionally, the study of DNA replication enhances our comprehension of cellular functions and identity. By breaking down the steps and mechanisms involved, it becomes possible to develop targeted therapies that can address genetic disorders or cancer. As such, this exploration provides essential insights not only for academia, but also for practical applications in healthcare.
Purpose of DNA Replication
DNA replication serves multiple purposes that are foundational to the continuation of life. Primarily, it ensures that every new cell has an exact copy of the DNA from the parental cell. This occurs during the S phase of the cell cycle and is paramount for growth and repair. Without DNA replication, multicellular organisms would not be able to grow or heal effectively.
Moreover, DNA replication plays a role in evolution. Through copying, variations can occur which can lead to genetic diversity. This diversity is essential for natural selection, allowing organisms to adapt to changing environments. Therefore, understanding how DNA replication operates provides insight into not only cellular biology but also broader evolutionary processes.
History and Discovery
The story of DNA replication is not just scientific; it is a tapestry of historical intrigue. The elucidation of the DNA structure by James Watson and Francis Crick in 1953 provided the foundation for understanding how genetic information is stored and transmitted. Their model of the double helix indicated a method of replication that was initially termed "semi-conservative." In this model, each strand serves as a template for creating a new complementary strand.
Further research, including pivotal experiments by Meselson and Stahl in the late 1950s, confirmed the semi-conservative nature of DNA replication. Their experiments demonstrated that each new DNA molecule consists of one old strand and one newly synthesized strand. This groundbreaking work illustrated that DNA replication was not a random event but followed a highly organized and regulated process.
The historical context of DNA replication has led to ongoing studies in molecular biology, genetics, and molecular medicine, which continue to unveil the complexities inherent in the replication process and the enzymes involved. This ever-evolving field holds immense promise in enhancing our understanding of life's molecular underpinnings.
Molecular Structure of DNA
The molecular structure of DNA serves as the cornerstone of understanding genetic replication. Knowledge of this structure is crucial for comprehending how genetic information is stored, replicated, and transmitted within an organism. DNA, or deoxyribonucleic acid, is a complex macromolecule that carries the hereditary instructions essential for the growth, development, functioning, and reproduction of all known living organisms and many viruses.
Double Helix Architecture
One of the most notable features of DNA is its double helix architecture. The term "double helix" describes the structure consisting of two long strands of nucleotides twisted around each other. These strands are held together by complementary base pairs, forming a secure helical configuration. This geometry is not just aesthetically pleasing; it is essential for the stability of genetic information. Each strand runs in opposite directions, a feature described as antiparallel orientation. This is critical during the replication process as it influences how the replication machinery interacts with these strands.
- The bases adenine, thymine, guanine, and cytosine provide the coding potential for genetic information.
- The hydrogen bonds between complementary bases—A with T and G with C—ensure that the two strands can separate during replication, allowing accurate copying of genetic information.
- The major and minor grooves between the two strands allow proteins to access the bases for essential processes like replication and transcription.
"The double helix structure of DNA not only protects genetic information but also facilitates the precise mechanism of replication and repairs."
This architecture underscores the importance of DNA replication. The process requires the separation of the two strands, which only works due to the stable yet flexible nature of the double helix. Any changes in this structure could have far-reaching consequences for genetic stability.
Nucleotide Composition
Nucleotides are the building blocks of DNA, and understanding their composition is vital for appreciating the complexities of DNA replication. Each nucleotide consists of three components: a phosphate group, a sugar molecule (deoxyribose), and a nitrogenous base. The arrangement of these nucleotides in a polymer chain forms the unique sequences that encode genetic information.
- The phosphate group connects the sugar of one nucleotide to another, forming a sugar-phosphate backbone.
- The order of nitrogenous bases—adenine, thymine, guanine, and cytosine—dictates the genetic code, influencing everything from physical traits to susceptibility to diseases.
The sequence variation among these nucleotides contributes to genetic diversity, which is fundamental for evolution. Accurate replication is crucial for maintaining this diversity while ensuring fidelity. Errors in nucleotide incorporation can lead to mutations, reshaping genetic traits across generations. Thus, this composition is essential not only for replication but also for preserving the integrity of the genetic lineage, making it a pivotal topic in the discussions of DNA replication.
Key Enzymes in DNA Replication
The process of DNA replication is critically dependent on several key enzymes that facilitate each step of this complex mechanism. These enzymes ensure the accurate and efficient duplication of the genetic material, a task that is vital for cellular function and inheritance. Understanding these enzymes provides insight into how replication occurs at a molecular level and highlights the potential implications of errors during the process.
DNA Polymerase
DNA polymerase is essential for synthesizing new DNA strands during replication. This enzyme catalyzes the addition of nucleotides to a growing DNA chain, using an existing strand as a template. There are several types of DNA polymerases, each with specific roles in prokaryotic and eukaryotic cells. In eukaryotes, DNA polymerase alpha is primarily involved in lagging strand synthesis, while DNA polymerase delta plays a crucial role in leading strand synthesis.
- Functions of DNA Polymerase include:
- Adding nucleotides complementary to the template strand
- Proofreading newly synthesized DNA for errors
- Ensuring the fidelity of DNA replication through correction mechanisms
The ability of DNA polymerase to correct mistakes—via its 3’ to 5’ exonuclease activity—enhances the accuracy of replication, thus maintaining genetic stability. Errors that are not corrected can lead to mutations, which may have significant biological consequences.
Helicase
Helicase is a critical enzyme involved in unwinding the double helix structure of DNA, which is necessary for replication. By breaking the hydrogen bonds between the nucleotide pairs, helicase separates the two strands of DNA, resulting in the formation of the replication fork. This action is vital, as the exposed strands serve as templates for the synthesis of new DNA.
- Key aspects of Helicase's function include:
- Unwinding the DNA helix
- Incorporating energy to facilitate strand separation
- Preventing the re-annealing of DNA strands during replication
A failure in helicase function can stall replication and lead to genomic instability. The activity of helicase is tightly regulated, as uncontrolled unwinding can cause excessive DNA damage and hinder cellular processes.
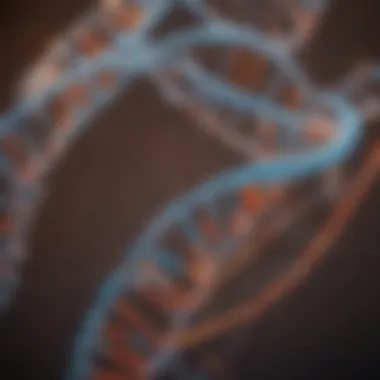
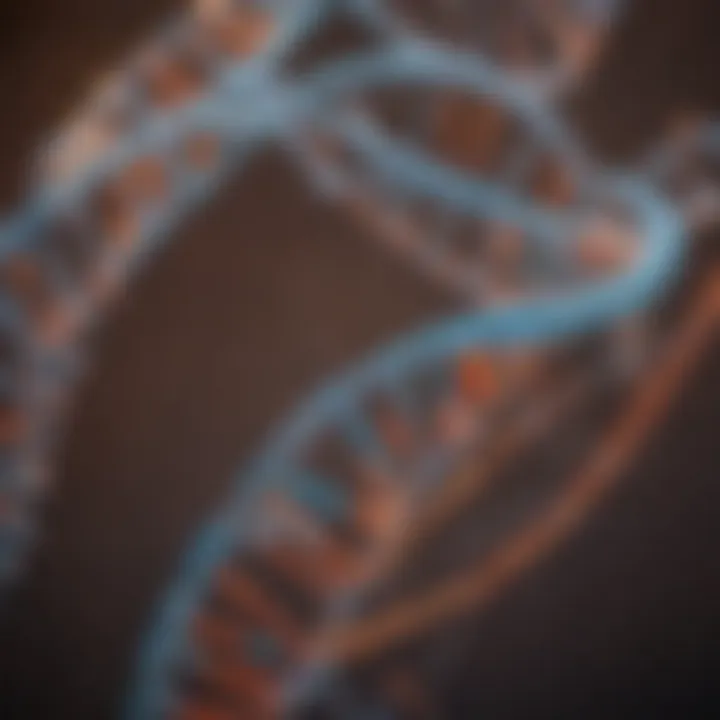
Ligase
DNA ligase is the enzyme responsible for joining Okazaki fragments during lagging strand synthesis. After DNA polymerase synthesizes short segments of DNA, ligase seals the nicks between these fragments, resulting in a continuous DNA strand. Without ligase, the replication process would be incomplete, leading to fragmented DNA and potential loss of genetic information.
- Notable functions of Ligase include:
- Sealing the phosphate-sugar backbone of DNA
- Facilitating efficient replication on the lagging strand
- Assisting in DNA repair processes
Overall, DNA ligase plays a crucial role in maintaining the integrity of the genetic material by ensuring that the newly formed DNA is cohesive and correctly structured.
"The nuanced interplay of these enzymes showcases the precision of molecular biology, where each component contributes to the larger narrative of life and genetic continuity."
Initiation of DNA Replication
The initiation of DNA replication marks a crucial phase in the cellular life cycle. This step is where the entire process of genetic duplication begins. Without proper initiation, the fidelity and efficiency of DNA replication can be significantly compromised, which can lead to mutations or cell death.
One of the primary elements in initiation is the recognition of specific sequences in the DNA known as the origin of replication. This sequence acts as a starting point where various proteins and enzymes gather to prepare the DNA for copying.
Origin of Replication
The origin of replication is a defined region on the DNA strand where the process begins. In eukaryotic cells, there are multiple origins of replication along each chromosome, allowing for multiple segments of the DNA to be copied simultaneously. This multiplexing of replication origins decreases the time required for cell division significantly.
Several proteins, including origin recognition complex (ORC), assemble at the origin. This assembly initiates the unwinding of the DNA strand, a critical early step in replication. Once the ORC is bound, it recruits additional proteins such as helicase, which plays a key role in separating the two strands of DNA. This makes the genetic code accessible for copying.
Formation of the Replication Fork
The formation of the replication fork is the next vital step following the identification of the origin of replication. As helicase unwinds the DNA double helix, two single strands of DNA are created, forming what is known as the replication fork. This fork resembles a Y-shape at the junction where the double strands separate, providing a site for the new DNA strands to be synthesized.
The dynamics of the replication fork are complex. Leading and lagging strands are synthesized differently due to the directionality of DNA polymerase. In simple terms, the leading strand is created continuously in the direction of unwinding, whereas the lagging strand is synthesized in short segments, known as Okazaki fragments.
The replication fork is not just a physical structure; it embodies the coordination of multiple enzymes and proteins to ensure that DNA replication occurs smoothly and accurately.
In summary, the initiation of DNA replication is a multi-faceted process that involves identifying the origin of replication and forming the replication fork. Understanding these processes lays the groundwork for comprehending how DNA replication is regulated and executed correctly within the cell.
Elongation Phase
The elongation phase of DNA replication is a critical stage that contributes to the overall fidelity and efficiency of the replication process. During this phase, newly synthesized DNA strands are formed, ensuring that each daughter cell receives an accurate copy of the genetic material. The elongation phase is highly regulated and involves several intricate mechanisms and key enzyme activities.
Leading Strand Synthesis
In the leading strand synthesis, DNA polymerase plays a pivotal role in extending the new DNA strand. This process occurs continuously in the 5' to 3' direction, taking advantage of the template strand that directs the synthesis of the complementary strand. The leading strand is synthesized rapidly because it follows the unwinding of the DNA double helix.
The action of helicase is essential here, as it unwinds the double helix ahead of the polymerase, creating a single-stranded template for replication. This synchronous action allows for a relatively efficient replication process and conserves time and energy for the cell. Importantly, various factors contribute to the fidelity of this synthesis, including the proofreading ability of DNA polymerase, which eliminates misincorporated nucleotides. This error correction ensures that genetic information is accurately copied and maintained.
Lagging Strand Synthesis
While leading strand synthesis is continuous, the lagging strand presents distinct challenges due to its discontinuous nature. Synthesis of the lagging strand occurs in short segments known as Okazaki fragments, which are initiated at multiple points along the template strand. Each fragment begins with a short RNA primer laid down by RNA primase. Once an Okazaki fragment is formed, DNA polymerase extends it until it reaches the beginning of the next fragment.
The lagging strand synthesis is more complex because it must occur in a direction opposite to the replication fork movement. This requires the coordination of multiple enzymes, like DNA ligase, which joins the Okazaki fragments together, completing the continuous strand. The necessity for this segmented synthesis demonstrates the overall intricacy involved in replication and highlights the adaptability of cells to ensure precise genetic transmission.
"Understanding the elongation phase reveals the delicate balance between speed and accuracy that cells must maintain during DNA replication."
Okazaki Fragments
Okazaki fragments play a crucial role in the process of DNA replication, particularly in the synthesis of the lagging strand. These short sequences of DNA, ranging from 100 to 200 nucleotides in length in eukaryotes, are essential for the replication machinery to overcome the inherent directional nature of DNA synthesis. On the leading strand, replication proceeds continuously, while the lagging strand is synthesized discontinuously. This leads to the formation of multiple Okazaki fragments, which must later be processed to create a continuous DNA strand.
Formation and Function
The formation of Okazaki fragments begins with the unwinding of the double helix by helicase, leading to the exposure of the single-stranded template. As DNA polymerase adds nucleotides to the growing DNA strand, it cannot synthesize in the 3' to 5' direction on the lagging strand. Instead, it synthesizes short bursts of DNA in the 5' to 3' direction, resulting in discrete pieces known as Okazaki fragments. Each of these fragments is later linked together through the action of DNA ligase.
The primary function of these fragments is to ensure that the entire lagging strand is replicated accurately and efficiently. Without Okazaki fragments, the replication process would be slower and less efficient, potentially leading to incomplete replication. Their existence highlights the complexity and precision of the DNA replication process, underscoring the necessity for coordinated action among various enzymes and proteins.
Role of RNA Primase
RNA primase is a vital enzyme in the synthesis of Okazaki fragments. It synthesizes short RNA primers that provide a starting point for DNA polymerase to extend and add deoxynucleotides. Because DNA polymerases can only add nucleotides to an existing strand, these primers are essential for initiating the replication of each Okazaki fragment. Typically, the primers are around 5 to 10 nucleotides long.
After the RNA primers have served their purpose, they must be removed to allow for the complete sealing of the DNA strand. The removal of these primers is another critical step in maintaining the integrity of the newly synthesized DNA. In eukaryotic cells, this task is accomplished by the combined action of RNase H, which degrades the RNA, and DNA polymerase, which fills in the resulting gaps with DNA nucleotides.
Overall, the process involving Okazaki fragments and the function of RNA primase illustrate the remarkable intricacies of DNA replication. These components emphasize how DNA synthesis is not merely about copying genetic information, but rather involves a complex interplay of enzymes and mechanisms that ensure fidelity and efficiency.
Termination of DNA Replication
The process of DNA replication reaches its conclusion with termination, a vital phase that ensures the proper duplication of genetic material. Understanding how termination occurs is crucial, as it plays a central role in maintaining genomic integrity. During the termination phase, the replication machinery must effectively manage the complete synthesis of the DNA strands while preventing potential errors that could lead to mutations or chromosomal abnormalities.
Completion of Replication Fork
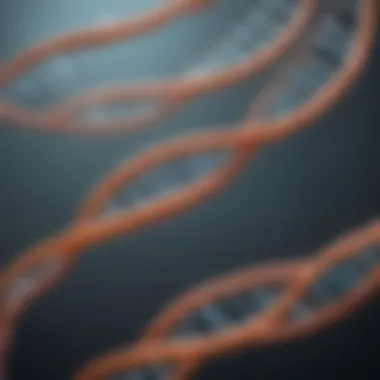
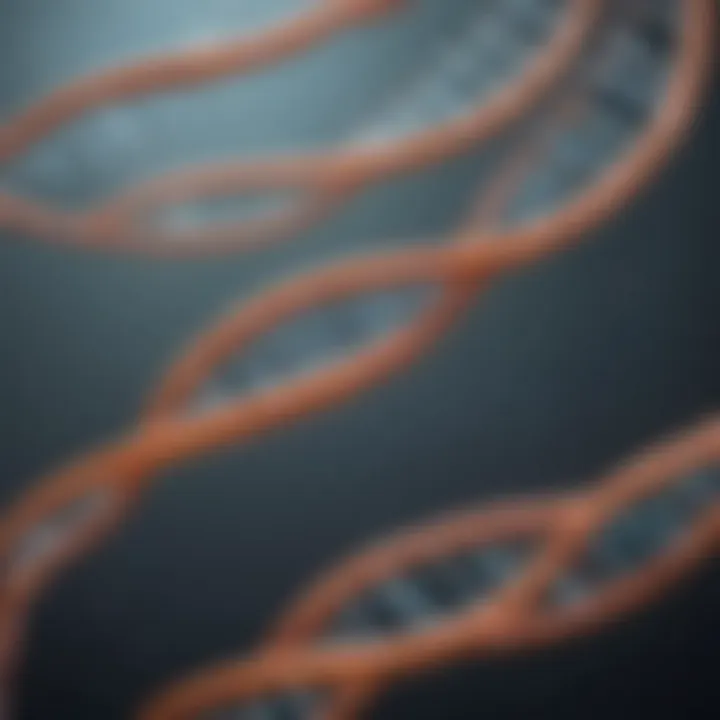
The replication fork is a dynamic structure that forms at the site of DNA unwinding and synthesis. By the time replication reaches its termination point, both strands of the DNA molecule must be fully synthesized.
- Each replication fork moves along the DNA template, copying the strand until the end of the template is reached.
- When replication forks from adjacent origins of replication meet, completion of the DNA molecule occurs. This is a critical moment, as it needs to ensure that all genetic information is accurately copied.
- Enzymatic interactions are essential during this phase. DNA polymerases help in finishing strand synthesis, often through mechanisms that ensure completing any unfinished segments of the lagging strand, which is especially important when nucleotides are not added in the correct order.
Understanding this completion process is paramount for researchers, as any disruption may lead to incomplete or error-prone replication.
Removal of RNA Primers
During DNA replication, RNA primers play a significant role in initiating the synthesis of new DNA strands. However, upon reaching the termination phase, these RNA primers need to be removed to finalize the newly synthesized DNA.
- RNA primase lays down short RNA segments that serve as starting points for DNA polymerase. Once replication is done, these segments pose a potential risk. If left intact, RNA fragments can interfere with the DNA structure and function.
- The removal of these primers primarily involves the action of the enzyme RNase H, which recognizes and degrades the RNA within the RNA-DNA hybrid regions. DNA polymerase then fills in the gaps left by the excised RNA.
- This meticulous removal process is essential for ensuring that DNA retains its stability and functionality after replication. The precise action of these enzymes allows the DNA strands to be completely free of RNA, thus preparing them for subsequent cellular processes.
By effectively managing the termination of DNA replication, cells safeguard their genetic material against potential discrepancies that could arise if RNA is not correctly removed.
Proofreading Mechanisms
The concept of proofreading mechanisms in DNA replication is crucial for maintaining genetic integrity. This aspect of molecular biology ensures that errors introduced during DNA synthesis are corrected. The fidelity of genetic information is vital for the survival of an organism. Any mistakes in DNA can lead to mutations, which may disrupt normal cellular functions or lead to diseases such as cancer. Therefore, understanding proofreading mechanisms is essential for appreciating how cells safeguard their genetic material.
Error Correction by DNA Polymerase
DNA polymerases possess inherent proofreading capabilities. These enzymes can identify and correct mismatched nucleotides during DNA synthesis. When an incorrect nucleotide is incorporated into the newly synthesized strand, the polymerase has a mechanism to recognize this anomaly. This recognition prompts the enzyme to retract slightly, allowing the wrong nucleotide to be excised. Once corrected, the polymerase can continue elongating the DNA strand effectively. This process is often referred to as 3' to 5' exonuclease activity. The advantage of this mechanism lies in its ability to prevent the proliferation of errors during replication.
Mismatch Repair Systems
Even with the error-correcting abilities of DNA polymerases, some mismatches can escape correction. This is where mismatch repair systems come into play. These systems act after DNA replication is complete. They identify and repair base pairing errors that were missed during the initial synthesis. Mismatch repair involves several proteins that recognize and bind to the incorrect base pair. Subsequently, they facilitate the removal of a section of the DNA strand containing the error. The gap is then filled in by DNA polymerase, followed by DNA ligase sealing the nick to restore continuity.
The efficiency of mismatch repair systems significantly enhances the overall fidelity of DNA replication, thereby reducing the mutation rate in organisms.
In summary, proofreading mechanisms in DNA replication encompass essential processes like the error correction by DNA polymerase and mismatch repair systems. These systems work collaboratively to ensure high fidelity in the genetic material transmitted from one generation to the next.
Regulation of DNA Replication
Regulation of DNA replication is a vital aspect of cellular function. It ensures that DNA is duplicated accurately and at the appropriate time. The tight control of this process helps maintain genomic stability and prevents issues that can lead to diseases like cancer. In this section, we will explore key elements in the regulation of DNA replication, its benefits, and the considerations necessary for understanding its critical role in biology.
DNA replication is intricately linked to the cell cycle. Each phase of the cell cycle has a designated timing for DNA replication to occur. This timing is crucial because any failure to replicate DNA accurately can result in mutations or incomplete copies of the genetic material. The regulatory mechanisms that control the timing and process of DNA replication interact with various cellular signals and checkpoints to maintain order, thereby safeguarding the integrity of genetic information.
Cell Cycle Phases and Replication Timing
During the cell cycle, DNA replication primarily occurs in the S phase. However, prior to the onset of this phase, cells must progress through the G1 phase. In G1, the environment is evaluated to ensure that conditions are suitable for replication. Nutrient availability and DNA integrity are crucial checks performed during this period. If all conditions are favorable, cells enter the S phase, where DNA replication is initiated.
The G2 phase serves as another checkpoint. During G2, the cell verifies that DNA replication has been completed successfully and prepares for mitosis. Any detected errors during replication may trigger repair mechanisms or lead to cell cycle arrest, preventing the cell from dividing until issues are resolved. This makes the regulation of DNA replication a crucial aspect not only for genetic fidelity but also for overall cell health.
Checkpoints and Repair Pathways
Cellular checkpoints serve as quality control mechanisms that monitor and regulate the integrity of the genetic material during replication. If errors are detected at any point in the cell cycle, checkpoints can halt progression, allowing for repairs or corrections. The important checkpoints are:
- G1/S checkpoint: Verifies if the DNA is undamaged and if the cell has the necessary energy and resources before entering the S phase.
- G2/M checkpoint: Ensures that all DNA has been accurately replicated and remains intact before the cell enters mitosis.
When DNA damage is detected, repair pathways are activated. These may include nucleotide excision repair and homologous recombination, depending on the nature and extent of the damage. This relationship between checkpoints and repair pathways underlines the significance of regulation in preventing replication errors.
"The regulation of DNA replication not only ensures fidelity but also is essential for maintaining stability across generations of cells."
Significance of DNA Replication
DNA replication is a cornerstone of cellular biology, serving multiple critical functions that are essential for life. This process not only contributes to genetic continuity but also plays a vital role in evolution and stability of genomes. Understanding the significance of DNA replication helps illuminate its multifaceted importance in both physiological and evolutionary contexts.
Implications for Genetic Stability
The most immediate implication of DNA replication is its role in maintaining genetic stability. Every time a cell divides, it must accurately duplicate its DNA. This precision ensures that each daughter cell inherits an exact copy of the genetic material present in the parent cell. If replication errors occur, they can lead to mutations. These mutations, if not corrected, may produce diverse effects, ranging from benign to highly detrimental.
The cellular mechanisms, such as proofreading by DNA polymerase and various repair pathways, function to minimize errors. These error-correction strategies are crucial for preserving the integrity of the genome. When they fail, the consequences can result in cancer and genetic disorders. Thus, reliable DNA replication is foundational for the health and stability of an organism’s genetic blueprint.
Role in Evolutionary Processes
Beyond its role in individual organisms, DNA replication plays a crucial part in evolutionary processes. Variation is the raw material for evolution, and mutations occurring during replication provide genetic diversity within populations. Such genetic variations can arise from replication errors or from exposure to environmental factors, leading to new traits that may offer an advantage in adaptation.
Over time, these variations can become fixed in a population through natural selection. For instance, beneficial mutations may lead to more successful offspring, guiding evolutionary trajectories. This process connects DNA replication directly with the grand narrative of life on earth, emphasizing its importance not merely as a biochemical reaction but as a driver of biodiversity.
In summary, the significance of DNA replication transcends the fundamental biological requirement of copying genetic information. It lays the groundwork for genetic stability while simultaneously sparking the evolutionary change that drives diversity. Recognizing these implications is essential for comprehending broader biological concepts and the processes that shape life.
Applications in Biotechnology
The significance of DNA replication in biotechnology cannot be overstated. This essential biological process serves as the foundation for various biotechnological applications, particularly those concerned with genetic manipulation and molecular biology research. Understanding how DNA replication works enables scientists and researchers to harness its mechanisms for innovative applications, ultimately benefiting fields such as medicine, agriculture, and forensic science.
One of the main areas where DNA replication is applied is in the development of diagnostic tools and therapeutic strategies. For instance, the ability to replicate specific DNA sequences through methods such as Polymerase Chain Reaction (PCR) has revolutionized diagnostics by allowing quick and precise identification of pathogens. Similarly, insights into DNA replication dynamics can enhance gene therapies by helping to manage gene delivery and expression in target cells.
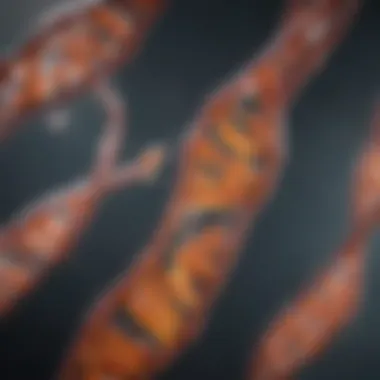
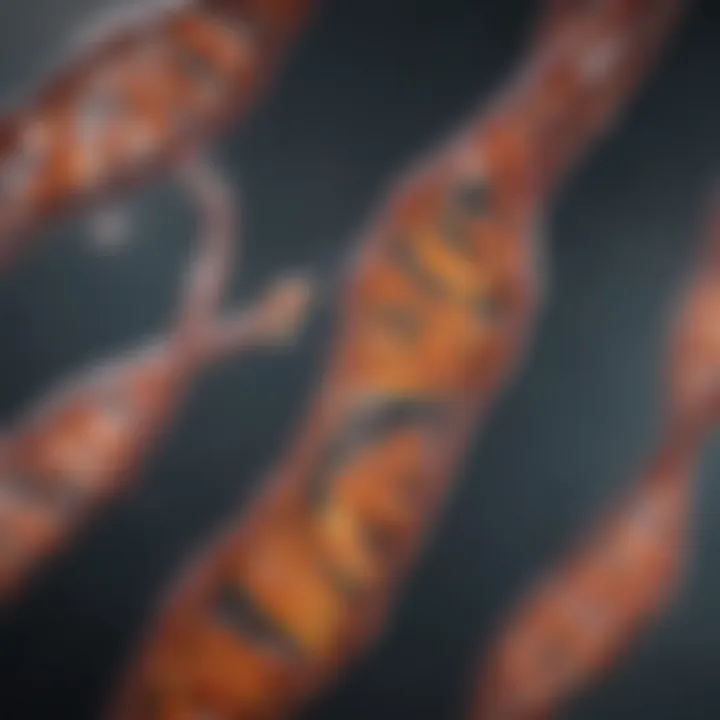
"PCR is a central technique in molecular biology, enabling the amplification of specific DNA sequences for analysis and experimentation."
By employing unique techniques for manipulating DNA, researchers can develop better bioengineering strategies, including the use of genetically modified organisms (GMOs). These GMOs can have enhanced traits, such as improved resistance to diseases or pests, which can be crucial for increasing crop yields and food security in an ever-growing population.
Additionally, understanding the replication processes can inform the production of recombinant proteins, which are essential for creating vaccines, hormones, and enzymes used in various therapeutic contexts. This knowledge assists in troubleshooting production issues, ensuring that the desired proteins are produced in sufficient quantities and quality.
However, there are several considerations that must be taken into account while utilizing DNA replication processes in biotechnological applications. Potential risks include issues related to ecological disruption when introducing genetically modified organisms into environments, as well as ethical concerns regarding genetic manipulation in humans and other species. Rigorous regulation and oversight are necessary to mitigate these risks.
Overall, the applications of DNA replication in biotechnology provide myriad benefits across various industries, while also demanding careful consideration of the associated ethical and societal implications.
Challenges in DNA Replication Studies
The study of DNA replication is vital for understanding fundamental biological processes. However, this area of research is fraught with challenges that make it complex and demanding. Several specific elements merit attention in discussions of DNA replication, as they highlight both the obstacles researchers face and the potential benefits of overcoming these issues.
Understanding Complex Interactions
DNA replication does not operate in isolation. It involves a multitude of proteins and enzymes working synchronously, often in a tightly regulated manner. Each component has a distinct role, but they also interact with each other in intricate ways. For example, DNA polymerase must coordinate with helicase to ensure that the DNA strands separate correctly before replication can occur. Additionally, the involvement of molecular chaperones can complicate the picture, as these proteins assist in folding other proteins properly during the replication process.
Understanding these complex interactions is crucial. It allows scientists to pinpoint where things may go awry and understand the full scope of DNA replication's physiological implications. When considering cancer research or genetic disorders, grasping the dynamics of these interactions can lead to significant advances in therapeutic approaches.
Research often employs various experimental techniques, including biochemical assays and high-resolution imaging. Still, clarifying these complex interactions requires innovative strategies and often, a reductionist approach that may oversimplify biologically accurate systems.
Technological Limitations
Despite advancements in technology, several limitations still hinder DNA replication studies. These constraints affect the precision of experiments and the data obtained, leading to interpretations that may not reflect the biological reality.
High-resolution imaging techniques, such as single-molecule fluorescence microscopy, offer insights into DNA replication dynamics but are constrained by resolution limits and photobleaching. Additionally, traditional biochemical assays may not capture the full range of in vivo conditions, causing researchers to rely on models that may not accurately depict the biological environment.
Moreover, the vast amount of data generated by modern sequencing technologies poses bioinformatics challenges. Analyzing this data requires sophisticated algorithms and computational tools, which may not always yield clear answers. The integration of various data types—genetic, epigenetic, and transcriptomic—adds layers of complexity, making it difficult to draw conclusive insights.
Despite these technological limitations, researchers are not at a total impasse. Continuous advancements in genomics, proteomics, and bioinformatics do provide a silver lining. By combining these technologies, scientists can explore new dimensions of DNA replication with enhanced clarity and depth.
"Understanding the challenges in DNA replication studies is essential for future advancements in genomics and therapeutic development."
In summary, while challenges exist, tackling them can provide critical insights into DNA replication processes. This knowledge can lead to breakthroughs that enhance our understanding and treatment of various diseases.
Future Directions in DNA Replication Research
Future directions in DNA replication research are critical for understanding how this fundamental process can be harnessed and manipulated in various contexts. As our comprehension of DNA and its functions continues to evolve, innovative approaches and technologies promise to enhance both basic and applied research. This section delves into emerging techniques in genomics and potential clinical applications, shedding light on why they are vital for advancing the field.
Emerging Techniques in Genomics
Recent advancements in genomic techniques have transformed our capabilities to study DNA replication. For instance, single-cell sequencing allows researchers to observe replication dynamics at the cellular level. This is significant because it reveals how different cells within a tissue may replicate their DNA under varying conditions.
Furthermore, CRISPR-Cas9 technology enables precise editing of genomic sequences, providing opportunities to study the effects of specific mutations on replication. Techniques like nanopore sequencing are revolutionizing the speed and accuracy of DNA analysis, making it feasible to unravel intricate replication mechanisms in real-time.
Another promising area is the development of high-throughput methods. These allow for the examination of large datasets to identify patterns in DNA replication across various organisms. By employing bioinformatics and machine learning, scientists can predict how alterations in DNA sequences might impact replication fidelity.
Potential Clinical Applications
The implications of understanding DNA replication extend into clinical settings, especially in the realm of personalized medicine. By decoding the mechanisms behind replication errors, researchers can identify genetic predispositions to diseases such as cancer. For example, studying the role of specific DNA polymerases in cancer can aid in developing targeted therapies that improve treatment outcomes.
In addition, better comprehension of DNA repair pathways may lead to interventions that enhance cellular repair mechanisms. This can be particularly relevant in age-related diseases and genetic disorders where replication errors contribute to cellular dysfunction.
Emerging techniques in drug development also benefit from insights into DNA replication. For instance, compounds that inhibit certain aspects of replication could serve as effective anti-cancer agents. Researchers are already looking into agents that target DNA replication proteins, potentially leading to breakthroughs in cancer therapeutics.
The End
The conclusion section serves as a pivotal element in this discussion of DNA replication. It synthesizes the multifaceted aspects of replication, emphasizing its significance in biology and genetics. By capturing the core concepts previously discussed, this section acts as a closing statement that highlights the importance of understanding DNA replication not only in basic science but also in applied fields.
In this article, various elements related to DNA replication were dissected. This includes the structure of DNA, the roles of essential enzymes during replication, and the intricate processes from initiation to termination. Such a comprehensive analysis allows researchers and students to appreciate the complexities of genetic fidelity.
Summary of Key Concepts
Key concepts surrounding DNA replication encompass several vital areas:
- Molecular Mechanics: The double helix structure of DNA is critical to understanding how replication occurs.
- Enzymatic Functions: Enzymes such as DNA polymerase, helicase, and ligase perform specific roles to ensure accurate DNA duplication.
- Replication Phases: The phases of initiation, elongation, and termination each require precise orchestration for genetic integrity.
- Proofreading Mechanisms: Error correction is essential for maintaining genetic stability, underscoring the importance of accuracy in DNA replication.
Importance of DNA Replication in Cellular Biology: At a fundamental level, DNA replication is vital for cell division and organismal growth, impacting everything from genetic traits to disease susceptibility.
Importance in Scientific Discourse
The relevance of DNA replication extends beyond mere computational or laboratory significance. It plays a crucial role in various quarters of evolutionary biology, genetic research, and medical science.
- Evolutionary Processes: Understanding how DNA replicates helps decipher evolutionary mechanisms, offering insight into genetic variation that drives evolution.
- Clinical Applications: Knowledge of replication processes paves the way for advances in gene therapy, cancer treatment, and regenerative medicine.
A well-rounded understanding of DNA replication enriches scientific dialogue by framing questions about genetic stability, disease, and therapeutic avenues. As we delve into the complexities and nuances of DNA replication, researchers can leverage this knowledge for innovations that benefit both scientific and medical fields.
"Grasping the intricacies of DNA replication unlocks numerous possibilities in genetics, with ramifications in health, evolution, and technology."
In summary, the conclusion of this article solidifies the relevance of DNA replication. It underscores the need for ongoing research and discourse in this essential biological process.